INTRODUCTION
Muscular dystrophies (MDs) comprise an extensive collection of hereditary conditions. These conditions are characterized by the gradual deterioration, wasting, and degeneration of muscles. These conditions vary in terms of inheritance patterns, onset times, and rates of muscle degeneration due to mutations affecting the key genes for muscle structure and function, thereby leading to this group of diseases. Among the notable forms of MD are congenital MD, Myotonic muscular dystrophy, Duchenne muscular dystrophy (DMD), and Becker muscular dystrophy (BMD), each of which possesses distinct genetic foundations and clinical presentations. The hallmark of these disorders is a progressive compromise of muscle integrity, which often results in significant morbidity and mortality, impacting both skeletal and cardiac muscles. Muscle degeneration in MDs is progressive and challenging, making life difficult for those affected. People with these conditions require extensive care and management to deal with the challenges posed by weakened muscles. Understanding the genetic and clinical aspects of MD types is critical for accurate diagnosis, treatment, and care for affected individuals.
OVERVIEW OF MDs
Types and genetic basis
MDs result from mutations in genes encoding proteins responsible for maintaining muscle cell stability, which leads to progressive muscle degeneration. For instance, DMD, which is caused by mutations in the dystrophin gene, is a particularly severe type of MD that mainly affects males. It occurs in approximately 1 out of every 3500 newborn males. On the other hand, BMD, which is the result of a mutation in the dystrophin gene, however, shows a less severe phenotype. Other types of MD are caused by mutations in genes such as those that encode laminin subunits (congenital muscular dystrophy) and myotonic dystrophy protein kinase (myotonic dystrophy). Each of these mutations contributes to specific clinical characteristics and the progression of the disease (Guiraud et al., 2015).
MD is a condition characterized by progressive weakness of voluntary muscles. This weakness typically begins in the proximal muscles of the hips, thighs, and shoulders, and impairs tasks like lifting objects, walking, climbing stairs, or raising arms overhead. In MD, affected muscles may experience atrophy, leading to a decline in strength and muscle mass. Early symptoms include frequent falls and walking difficulties. Some forms of MD may result in joint contractures, restricting the joint range of motion. Additionally, respiratory insufficiency may occur due to the gradual deterioration of respiratory muscles, necessitating respiratory support. In particular, DMD can affect the myocardium and induce heart arrhythmias. Certain MD variants, like Duchenne and Becker muscular dystrophies, may cause cognitive or behavioral difficulties. Motor milestone attainment delays may occur in infants. During periods of prolonged inactivity or physical exertion, MD patients may experience muscle pain, cramps, and stiffness.
Current treatment and challenges for MD
The treatment for MD involves varied therapeutic strategies due to distinct subtypes of MD exhibiting distinct genetic origins and disease courses. DMD and spinal muscular atrophy (SMA) (Guiraud et al., 2015) are two forms of MD caused by mutations in genes. DMD has seen significant advancements in disease-modifying therapies, including exon-skipping therapies like Eteplirsen and Golodirsen, which slow disease progression (Roberts et al., 2023). SMA, while not strictly a form of MD, shares similarities in progressive muscle weakness, with treatments like Nusinersen and Onasemnogene abeparvovec revolutionizing management (Mercuri et al., 2020). Limb-girdle muscular dystrophies (LGMDs) are a group of genetic disorders characterized by progressive muscle weakness and atrophy, primarily affecting the limb and shoulder girdle muscles. Various subtypes exist, each caused by mutations in different genes, such as sarcoglycans, dysferlin, and calpain leading to predominant proximal muscle weakness, which can be seen in two or more unrelated families. Till now only symptomatic treatments are available; however, the LGMD treatment focuses on the root cause rather than its symptoms. Various techniques, including stem cell transplantation, exon-skipping, gene delivery, RNAi, and gene editing, are under investigation for treatment (Bouchard and Tremblay, 2023). Gene therapy is a promising approach to treating various forms of MD by delivering functional copies of defective genes or correcting mutations. In LGMD, gene therapy trials are underway targeting specific gene defects, showcasing the potential of this approach in managing MD. Current trials focus on LGMD subtypes, restoring missing proteins, delivering functional dysferlin, and using gene editing techniques like clustered regularly interspaced short palindromic repeats (CRISPER)-Cas9 (Chu and Moran, 2018). However, gene therapy faces challenges in immune response, efficient delivery, and combining with other therapies for synergistic benefits and enhanced treatment outcomes (Theadom et al., 2015; Georganopoulou et al., 2021). However, in gene therapy for MD, immune responses include the body’s recognition of the viral vectors as foreign entities which results in subsequent activation of an immune reaction. This may destroy the vector before the delivery of the biologically active gene. Furthermore, the presence of pre-existing immunity to the vector might completely hinder the delivery of genes. These reactions frequently affect clinical results, requiring the use of techniques such as immunosuppression or the production of less immunogenic vectors (Shirley et al., 2020).
Stem cell therapies, including mesenchymal stem cells (MSCs) and induced pluripotent stem cells (iPSCs), are being explored for their potential in managing MD. MSCs, found in various tissues, can differentiate into muscle cells, adipocytes, and bone cells (Bagno et al., 2022). iPSCs, generated by reprogramming adult cells, can differentiate into any cell type in the body, making them valuable for regenerative medicine (Moradi et al., 2019). These therapies aim to restore muscle function and regenerate damaged muscle tissue, offering a novel approach to managing MD. Pre-clinical studies have shown the therapeutic potential of MSCs in improving muscle function, reducing inflammation, and enhancing regeneration. However, challenges such as tumorigenicity, efficient differentiation protocols, and scalability need to be addressed. Clinical trials are ongoing to evaluate the safety and efficacy of these therapies (Berebichez-Fridman and Montero-Olvera, 2018; Zhou and Shi, 2023). Economic, scientific, and clinical factors all contribute to the difficulty of treating MD. The cost and accessibility of advanced therapies, such as gene therapies and biologics, limit the widespread adoption of these therapies. Additionally, ensuring the long-term efficacy and safety of novel treatments remains a challenge, especially in progressive diseases like MD where sustained therapeutic effects are crucial. Furthermore, the wide range of patients necessitates personalized treatment approaches. Even though advances in genomics and molecular diagnostics enable precision medicine, implementation remains difficult. Studies show that combination therapies, such as gene therapy and physical therapy, may optimize outcomes and improve the quality of life for MD patients (Lovering et al., 2005).
Natural compounds as potential therapies for MD
Historically, natural products have played a pivotal role in pharmacotherapy in the treatment of various diseases. Despite the technical obstacles pertaining to characterization, optimization, screening, and isolation, which pose difficulties for drug discovery, they are most recommended for their least side effects. Advances in microbial culture, genome mining, and engineering techniques, as well as technological and scientific developments such as improved analytical tools, are addressing these challenges and creating new opportunities (Atanasov et al., 2021). Additionally, the marine environment is filled with species that have adapted to harsh conditions; their ability to produce biotechnologically useful compounds has increased interest in marine organisms. Research has demonstrated that secondary metabolites produced by marine bacteria and fungi possess intricate and specific chemical structures, which could potentially serve as precursors to the development of new pharmaceuticals or compounds. Marine natural products exhibit a wide range of bioactivities that are of industrial importance, such as antimicrobial, antiviral, neurodegenerative, anticancer, and anti-inflammatory properties (Karthikeyan et al., 2022). There are numerous drug resources in nature that require identification and purification to be utilized as essential biologics in contemporary medicine. Pharmaceuticals are classified into three distinct groups: small bio-molecules, plant-derived biologics, and phytopharmaceutical drugs, a more recent classification. As a result of the concept of phytopharmaceutical drugs, which states that natural products from diverse sources can interact with multiple human physiological targets, phytopharmaceutical drugs have been developed (Nasim et al., 2022).
Different types of MDs and their genetic causes
DMD is a severe, progressive, muscle-wasting disorder primarily affecting males. It affects approximately 1 in 5000 live male births. Symptoms usually appear between the ages of 2-3 years, with the majority of patients requiring a wheelchair by the age of 10-12 years (Aartsma-Rus et al., 2006). Patients who are between the ages of 20 and 40 years typically pass away from cardiac or respiratory failure despite receiving optimal care. DMD is an X-linked recessive disorder that affects males (Landrum Peay et al., 2019). Female carriers may experience mild symptoms or develop dilated cardiomyopathy (Bez Batti Angulski et al., 2023). The absence of functional dystrophin in DMD causes fragile muscle membranes that are vulnerable to damage during muscle contraction, resulting in pathological changes such as inflammation, fibrosis, and muscle tissue loss. Dystrophin is essential for maintaining muscle cell membrane stability, and DMD mutations impair this function, resulting in muscle cell damage and progressive weakness. Cardiac complications, including dilated cardiomyopathy, arrhythmias, and heart failure, are common and often fatal in DMD patients. Respiratory muscles, particularly the diaphragm, are also affected, leading to ventilatory insufficiency. Cognitive and neurobehavioral disorders may also occur due to the lack of dystrophin in the brain, resulting in lower intelligence quotient (IQ), learning disabilities, and an increased risk of conditions like attention deficit hyperactivity disorder and autism spectrum disorder (ADHD). Treatment and prognosis for DMD patients include advances in care and management, but there is currently no cure (Budzynska et al., 2024).
Pathophysiological mechanisms
Instability of membrane
In normal muscle, the sarcolemma forms a stable and continuous surface that protects the muscle fibers from injury. However, with the help of the dystrophin–glycoprotein complex (DGC) and the integrin complex, the sarcolemma forms a well-knitted two-dimensional network. In DMD, the missing dystrophin, and, hence, lacking the DGC, means that there is a disconnection between the intracellular and the extracellular matrix (ECM) and, therefore, the structure of the sarcolemma is destroyed, making it easily injured (Guiraud et al., 2015). Injury translates into muscle damage, which is observed as the breaking of the sarcolemma, the high serum creatine kinase (CK), and the large protein-like albumin uptakes (Allen et al., 2016). Repeatedly stretching muscles for extended periods is more concerning as it increases the risk of muscle tearing (Stedman et al., 1991).
Calcium dysregulation
In DMD, membrane instability leads to an increased amount of intracellular calcium, which disrupts calcium homeostasis. This increased calcium may enter muscle fibers both from the tears of the sarcolemma and through numerous calcium channels. The increased calcium, through activation of negative signaling pathways including calmodulin, calpains, and dysferlin, impairs muscle function and its repair (Mareedu et al., 2021). Sarcoplasmic reticulum dysfunction adds to the dysregulation of calcium, and its interplay with reactive oxygen species (ROS) causes the situation to worsen (Duan et al., 2021), Figure 1.
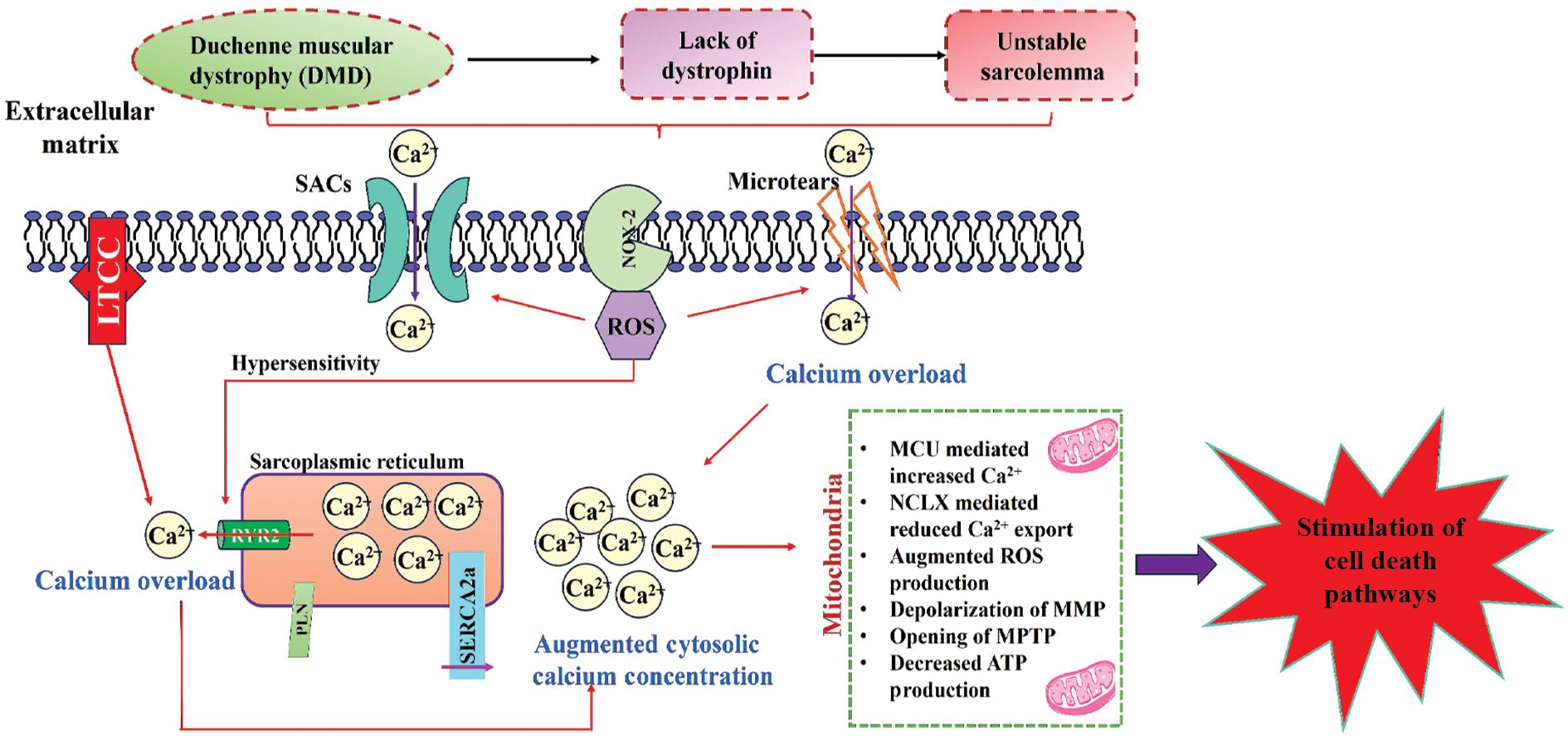
Representation of excitation–contraction coupling in cardiac myocytes. Normal excitation–contraction coupling in cardiac myocytes occurs when membrane depolarization causes a small calcium influx through the LTCC, which then releases more calcium from the SR via RyR2. Calcium binds to myofilaments, contracting myocytes. Calcium is reabsorbed into the SR by Serca2a or expelled through the Na+/Ca2+ exchanger during relaxation. Abbreviations: ATP, adenosine triphosphate; LTCC, L-type calcium channel; MCU, mitochondrial calcium uniporter; MMp, mitochondrial membrane potential; MPTP, mitochondrial permeability transition pore; NCLX, mitochondrial sodium/calcium exchanger; ROS, reactive oxygen species; RyR2, ryanodine receptor 2; SAC, stretch-activated channels; SERCA, sarcoplasmic/endoplasmic reticulum calcium ATPase; SR, sarcoplasmic reticulum.
Inflammation
A characteristic of DMD is inflammation, which aids in the disease’s progress and appears before clinical symptoms. Early immune cell infiltration, which includes T and macrophage infiltration, is linked to persistent inflammation and fibrosis. Damage-associated molecular patterns are continuously released as a result of membrane instability, which triggers immunological responses (Evans et al., 2009; Henriques-Pons et al., 2014). Induction of major histocompatibility complex expression on muscle fibers by pro-inflammatory cytokines further weakens immune privilege. Adipose tissue accumulation, fibrosis, and impaired regeneration are the outcomes of chronic inflammation (Wehling et al., 2001).
Mitochondrial dysfunction
DMD interferes with the homeostasis of mitochondria, from which mitochondrial dysfunction emerges. Opening of the permeability transition pore is caused by mitochondrial Ca2+ overload, leading to reduced adenosine triphosphate (ATP) synthesis. Structural abnormalities like reduced size and sparse cristae can be observed in the mitochondria of dystrophic muscles (Turner et al., 1988). Mitochondrial dysfunction thus arises, leading to myofiber necrosis with associated reduced mitochondrial density and respiration, impairing the energy production of muscles (Turner et al., 1988).
Dysregulation of reactive oxygen and nitrogen species
The generation of ROS in excess, which overcomes the antioxidant defenses in muscle, is a feature of DMD that causes oxidative damage (Reid et al., 1992). Significant sources of ROS within the dystrophic muscle are mainly mitochondria, especially complexes I and III. The higher levels of ROS induce a disorder in myofilament Ca2+ sensitivity and cross-bridge kinetics, which further results in the functional impairment of muscle contraction. This increase in NADPH oxidase 2 and delocalized neuronal nitric oxide synthase activity causes an increase in ROS production, increasing muscle damage. Such oxidative stress can be temporary due to the ROS-buffering capacity without long-term damage being avoided (Chance et al., 1979; Zhao et al., 2015).
Fibrosis
The primary characteristic of DMD is fibrosis, or the excessive production of connective tissue. Fibrosis is caused by recurrent cycles of injury and regeneration that impair muscle function. Myostatin, transforming growth factor-beta (TGF-β), and other factors increase the production of ECM, which in turn causes an increase in muscle stiffness. Early fibrosis treatment approaches seek to maintain muscle mass and improve regenerative ability (Lieber and Ward, 2013; Kharraz et al., 2014).
BMD is a rare genetic disorder caused by mutations in the dystrophin gene that causes progressive muscle degeneration, particularly in the lower limbs, and frequently results in heart failure. Unlike DMD, BMD is less severe and develops later, typically between the ages of 5 and 60 years. BMD predominantly affects males due to its X-linked inheritance pattern, with a prevalence less than that of DMD (Fong et al., 1990). BMD is caused by in-frame mutations in the dystrophin gene, which results in a reduced or dysfunctional dystrophin protein. Dystrophin is essential for maintaining muscle cell integrity; without adequate functional dystrophin, muscle cells become more susceptible to damage during contraction, resulting in progressive muscle weakness (Lai et al., 2009). BMD symptoms include progressive muscle weakness, dilated cardiomyopathy, cognitive dysfunction, growth impairment, and orthopedic complications. Its diagnosis involves genetic testing and immunohistochemical analysis, while differential diagnoses include other myopathies (Shrestha and Munakomi, 2024). Currently, there is no cure for BMD, but management focuses on supportive and rehabilitative care to maintain muscle function and improve quality of life. Corticosteroids, cardiovascular management, and rehabilitation therapy are essential for maintaining mobility and function. Emerging therapies, such as gene therapy and vamorolone, are being investigated. The prognosis for BMD patients is generally more favorable than those with DMD, with life expectancy ranging between 40 and 50 years. Effective management requires a multidisciplinary approach involving primary care physicians, geneticists, cardiologists, pulmonologists, and therapists (Angelini et al., 2019).
LGMD is a class of hereditary myopathies distinguished by proximal muscle weakness in the pelvic and shoulder girdles. They can appear at any age and fall into two categories: LGMD1 (autosomal dominant) and LGMD2 (autosomal recessive) (Angelini, 2020). LGMDs are difficult to diagnose because of their diverse clinical presentations and overlapping features. An algorithmic approach that takes into account weakness, inheritance patterns, and specific clinical or muscle biopsy features helps to narrow down the differential diagnoses. Genetic testing is critical in confirming diagnoses, guiding prognosis, developing appropriate management strategies, and facilitating genetic counseling (Nigro and Savarese, 2014). The therapeutic options for LGMDs are limited, with ongoing research into gene therapy, myoblast transplantation, and targeted therapies. Corticosteroids are currently recommended in specific subtypes, whereas growth hormone therapy and endurance training have yielded mixed results across LGMD subtypes (Simonds, 2006). Gene therapy trials targeting specific gene defects have shown promise but need to be further evaluated. Accurate genetic diagnosis is critical for confirming LGMDs, predicting outcomes, planning treatments, providing genetic counseling, and optimizing healthcare resource utilization. Clinicians should use an algorithmic approach that combines clinical assessment, genetic testing, and multidisciplinary management to provide comprehensive care to patients with LGMDs (Murphy and Straub, 2015).
Facioscapulohumeral muscular dystrophy (FSHD) is a neuromuscular disorder that causes progressive muscle weakness and atrophy, primarily in the face, shoulder girdle, and upper arms. It is the third most common MD, after DMD and myotonic dystrophy, with a prevalence of approximately 1:15,000 (Flanigan et al., 2001). FSHD is classified into two types: FSHD1 and FSHD2. FSHD1 causes DNA hypomethylation on both 4q35 alleles, which is usually caused by mutations in the SMCHD1 or DNMT3B genes. Both rely on the same downstream mechanism, which involves the toxic expression of the DUX4 protein in muscle (Schatzl et al., 2021). Patients with FSHD can present at any age, and the disease progresses slowly. The most common symptoms are facial and shoulder muscle weakness, scapular winging, foot drop, and abdominal muscle weakness. Pain and fatigue are frequently reported. Respiratory involvement can occur in approximately 10% of patients, potentially leading to restrictive lung disease. Individuals’ disease severity and progression vary greatly, with earlier onset and larger D4Z4 contractions being associated with a more severe disease (Tihaya et al., 2023). Currently, there are no disease-modifying treatments for FSHD, and ongoing research is aimed at understanding the molecular mechanisms underlying the condition and developing targeted therapies. Animal models have been instrumental in studying FSHD pathology and testing potential treatments (Schatzl et al., 2021), as presented in Table 1.
Comparative overview of MD types and characteristics.
MD type | DMD | BMD | LGMD | FSHD |
---|---|---|---|---|
Genetic cause | Mutations in the DMD gene, absent dystrophin | Mutations in the DMD gene, partially functional dystrophin | Mutations in various genes (sarcoglycans, dysferlin) | Contraction of D4Z4 repeats, aberrant DUX4 expression |
Clinical manifestations | Early onset, rapid muscle weakness, loss of ambulation | Later onset, slower progression, variable severity | Proximal muscle weakness, variable onset/severity | Facial/shoulder weakness, variable progression |
Disease progression | Rapid deterioration, respiratory/cardiac complications | Slower than DMD, variable course | Varies widely by subtype; slow to significant disability | Slow and variable; episodic exacerbations |
Prognosis | Historically poor; improved with therapies | Variable; milder than DMD | Highly variable; depends on subtype | Variable; emerging therapies may improve outcomes |
Abbreviations: BMD, Becker muscular dystrophy; DMD, Duchenne muscular dystrophy; FSHD, facioscapulohumeral muscular dystrophy; LGMD, limb-girdle muscular dystrophy; MD, muscular dystrophy.
Distal muscular dystrophy: molecular pathophysiology
Distal dystrophies are primary muscle disorders that are inherited and are distinguished by progressive weakness and atrophy in the hands, forearm, lower legs, or feet. Though categorized as MDs, the molecular genetic background now encompasses nearly 20 distinct disorders, making differential diagnosis both more challenging and easier (Udd, 2011). Distal phenotypes need to be distinguished from neurogenic conditions like chronic SMA and Charcot–Marie–Tooth disease. Certain conditions advance to affect the proximal muscle, whereas others stay distal for the duration of the patient’s life. Distal dystrophies are pathological conditions characterized by a progressive loss and degeneration of muscle fibers, which are replaced by fatty and fibrous connective tissue (Dimachkie and Barohn, 2014). Sarcomeric proteins are encoded by several genes implicated in distal dystrophies; however, the genetic pathways resulting in the preferential activation of distal muscles are still unclear (Udd, 2007), Figure 2. Each type of distal muscular dystrophy is covered in detail in this overview, along with its clinical manifestations, pathological characteristics, genetic foundations, and implications for diagnosis and treatment.
Laing early onset distal myopathy (MPD1) is a condition affecting the anterior lower leg muscles, typically affecting individuals aged 1 to 25 years. It often results in progressive weakness and atrophy, often accompanied by type 1 fiber atrophy in the tibialis anterior muscle. The disorder is inherited through an autosomal dominant pattern, with mutations in the myosin MYH7 gene (Meredith et al., 2004).
Distal nebulin myopathy is a condition affecting the anterior lower leg muscles, typically occurring between 2 and 15 years of age. It can cause progressive weakness without nemaline rods on light microscopy. The disease is inherited autosomally recessively, with mutations in the nebulin NEB gene on chromosome 2q21 (Fisher et al., 2022).
Udd distal myopathy (TMD) is a condition affecting the anterior lower leg muscles, typically presenting after 35 years of age. It can cause progressive weakness and dystrophic changes with rimmed vacuoles. Muscle biopsy may reveal these changes. The disorder is genetically inherited through mutations in the titin TTN gene (Udd and Hackman, 2020).
Telethoninopathy is an autosomal recessive limb-girdle muscular dystrophy type 2G (LGMD2G) caused by mutations in the TCAP gene, which encodes the sarcomeric protein telethonin (Moreira et al., 2000). This protein is found in skeletal muscle, the heart, and gastrointestinal smooth muscle, and is essential for sarcomeric assembly, sarcomere–membrane interactions, and stretch sensing. The clinical presentation of LGMD2G is consistent, typically involving early onset, limb-girdle weakness that predominantly affects the lower limbs. LGMD2G symptoms generally emerge during the second decade of life, although there is also a described congenital form. Conditions related to TCAP mutations include both dilated and hypertrophic cardiomyopathy, as well as isolated intestinal pseudo-obstruction due to altered telethonin function (Olivé et al., 2008). Most patients with telethonin deficiency are of Brazilian origin, but some have recently been described as Asian and European ancestry. Muscle biopsy may reveal rimmed vacuoles. The disorder is inherited in an autosomal recessive manner, with mutations identified in the telethonin gene located on chromosome 17q (Mazzone et al., 2008).
Desminopathy, also known as myofibrillar myopathy (MFM), is a pathological disorder characterized by the accumulation of myofibrillar degradation products and the expression of multiple proteins, including desmin, αB-crystallin (αBC), dystrophin, and congophilic amyloid material. The condition was first proposed in 1996 and has been linked to dominant mutations in desmin and αBC in some patients. MFM is morphologically distinct but genetically heterogeneous, with further research likely coming from linkage studies or systematic searches for mutations in proteins supporting the Z-disk network. It typically manifests between 15 and 40 years of age, affecting the distal leg and forearm muscles, often accompanied by cardiomyopathy (Selcen et al., 2004). Muscle biopsy may reveal dystrophic changes and desmin accumulation, with genetics involving autosomal dominant inheritance of mutations in the desmin gene on chromosome 2q35 (Udd, 2007).
Distal myotilinopathy (MFM) is a condition affecting the lower legs and hands, typically affecting individuals aged 40-60 years. It presents with progressive weakness, rimmed and non-rimmed vacuoles, and dark structures. Mutations in the myotilin gene (MYOT) have been linked to variable syndromes like LGMD1A and a subgroup of MFM (Olive et al., 2005). Muscle biopsy may reveal these features. The disorder has both autosomal dominant and sporadic inheritance patterns, with mutations in the myotilin gene on chromosome 5q31 (Feit et al., 1998).
Zaspopathy, also known as MFM, is a condition affecting the lower legs and hands, typically affecting individuals aged 40-60 years. It is associated with mutations in titin, dysferlin, GNE, desmin, and myosin. Recent research suggests that patients with MFM have a more distal muscle phenotype, with some potentially having mutations in myotilin, ZASP, filamin C, desmin, and αBC. The disorder is caused by the ZASP mutation A165V. Muscle biopsy may reveal rimmed and non-rimmed vacuoles, dark structures, and desmin-positive aggregates. Zaspopathy follows both autosomal dominant and sporadic inheritance patterns, with mutations identified in the ZASP gene on chromosome 10q (Griggs et al., 2007).
Distal myopathy with vocal cord and pharyngeal signs (MPD2) is a unique condition inherited as an autosomal dominant trait, first reported in a Caucasian family from Southern Tennessee (Feit et al., 1998). The disease typically presents between 35 and 60 years of age, characterized by asymmetric weakness in the lower legs and hands, often accompanied by dysphonia. Patients may exhibit rimmed vacuoles. Serum CK levels are normal to mildly elevated, and no nuclear inclusions were found. The disorder follows an autosomal dominant inheritance pattern, with mutations identified in genes located on chromosome 5q31. Muscle biopsy may reveal rimmed vacuoles (Malicdan and Nonaka, 2008).
Distal myopathy with rimmed vacuoles (DMRV) is an autosomal recessive muscle disorder affecting young adults, like hereditary inclusion body myopathy (HIBM). Initially described in Japan and Iran, DMRV and HIBM are now recognized as the same disease. DMRV and HIBM are caused by mutations in the GNE gene, which encodes a bifunctional protein with two enzymatic activities: UDP-GlcNAc2-epimerase (GNE) and ManNAc kinase. The disorder typically presents between 15 and 30 years of age, primarily affecting the anterior lower leg muscles (Nishino et al., 2002). Patients may demonstrate dystrophic changes with rimmed vacuoles. The disorder follows an autosomal recessive inheritance pattern, with mutations identified in the GNE gene located on chromosome 9p1-q1 (Nishino et al., 2005).
Welander distal myopathy (WDM) is a late-onset condition with an autosomal dominant inheritance. Symptoms of WDM initially manifest in the hands and progressively impact the distal muscles of the lower limbs, particularly targeting the long extensors of the hands and feet. CK levels are typically normal or only mildly increased, and the condition does not involve the heart. Additionally, the neurophysiological assessments reveal both myopathic and neuropathic characteristics, while histopathological analysis predominantly shows myopathic changes, including the presence of rimmed vacuoles. When manifesting after 40, patients may exhibit dystrophic changes with rimmed vacuoles (Borg et al., 1998). This disorder follows an autosomal dominant inheritance pattern, with mutations identified in genes located on chromosome 2p13 (Åhlberg et al., 1999), as shown in Table 2.
Characteristics of distal myopathies, including their OMIM numbers, onset age, CK levels, muscle pathology, and genetic factors.
Type | OMIM # | Onset | CK | Muscle pathology | Inheritance | Gene/location |
---|---|---|---|---|---|---|
Laing early onset distal myopathy (MPD1) | #160500 | 1-25 | 1-8× | Type 1 fiber atrophy in TA muscle, (no) vacuoles | AD | Myosin MYH7, 14q |
Distal nebulin myopathy | - | 2-15 | 1-3× | No nemaline rods on light microscopy, no vacuoles | AR | Nebulin NEB, 2q21 |
Udd distal myopathy (TMD) | #600334 | >35 | 1-4× | Dystrophic, rimmed vacuoles | AD | Titin TTN, 2q31 |
Distal onset in telethoninopathy | - | Early | 3-10× | Rimmed vacuoles | AR | Telethonin 17q |
Desminopathy (MFM) | #601419 | 15-40 | 1-3× | Dystrophic, rimmed vacuoles + desmin bodies | AD | Desmin 2q35 |
Distal myotilinopathy (MFM) | #609200 | 40-60 | 1-3× | Rimmed and non-rimmed vacuoles, GTC dark and hyaline | AD and sporadic | Myotilin 5q31 |
Zaspopathy (MFM) | #609452 | 40-60 | 1-3× | Rimmed and non-rimmed vacuoles, GTC dark and hyaline | AD and sporadic | ZASP 10q |
Distal MFM | #608810 | Adult | - | + desmin granulofilamentous | AD | αB-crystallin 11q |
Distal caveolinopathy | #601253 | Early | 3-10× | Reduced caveolin-3 | AD | CAV3 |
MM | #254130 | 15-30 | 10-100× | Dystrophic | AR | Dysferlin 2p13 |
Nonaka distal myopathy (DMRV/HIBM) | #605820 | 15-30 | 1-5× | Rimmed vacuoles | AR | GNE 9p1-q1 |
Welander distal myopathy | #604454 | >40 | 1-4× | Dystrophic, rimmed vacuoles | AD | 2p13 |
Distal myopathy with vocal cord and pharyngeal signs (MPD2) | #606070 | 35-60 | 1-8× | Rimmed vacuoles | AD | 5q31 |
Distal myopathy with pes cavus and areflexia | #601846 | 15-50 | 2-6× | Dystrophic, rimmed vacuoles | AD | 19p13 |
Juvenile-adult onset distal myopathy | - | 10-40 | 1-2× | Myopathic-dystrophic | AD | 12 genetic loci excluded |
Adult onset distal myopathy (MPD3) | - | >30 | 1-4× | Dystrophic, rimmed vacuoles, + eosinophilic inclusions | AD | 8p-q and 12q linked |
MM-like non-dysferlinopathy | - | 25-50 | 1-10× | Myopathic-dystrophic | AR and sporadic | Some 10q |
Abbreviations: AD, autosomal dominant; AR, autosomal recessive; CK, creatine kinase; DMRV, distal myopathy with rimmed vacuoles; HIBM, hereditary inclusion body myopathy; MFM, myofibrillar myopathy; MM, Miyoshi myopathy.
Natural compounds with potential therapeutic benefits for MD
Phytomedicine is a therapeutic approach that utilizes bioactive compounds derived from plants, such as polyphenols, flavonoids, terpenoids, and other phytochemical classes that possesses anti-inflammatory, analgesic, and chondroprotective properties. Several studies have identified novel phytochemicals with promising therapeutic potential in managing musculoskeletal disorders (MSDs) by targeting multiple pathways involved in inflammation and tissue repair. Advanced analytical techniques, such as metabolomics and proteomics, have enabled researchers to elucidate the molecular mechanisms underlying the therapeutic effects of phytochemicals on MSDs (Kantasrila et al., 2020). The historical presence of phytomedicine in ancient medical systems, such as Traditional Chinese Medicine, Unani, Ayurveda, and indigenous healing practices, is extensive (Islam, 2018). Medicinal plants have been used for centuries in many cultures around the world to promote musculoskeletal health, minimize inflammation, and ease pain. The therapeutic potential of plant-derived remedies in managing MSDs is emphasized by this long-standing tradition (Pan et al., 2014). Complex mixtures of bioactive compounds found in herbal remedies frequently have multi-targeted effects on the underlying pathophysiology of MSDs. Phytomedicine can modulate several pathways involved in inflammation, oxidative stress, cartilage degradation, and tissue repair, in contrast to single-target pharmaceutical drugs (Vaou et al., 2022). The multifaceted nature of MSDs is addressed by this comprehensive strategy, which could have synergistic therapeutic effects (Chandrasekara and Shahidi, 2018). Compared to synthetic drugs, herbal medicines have a more favorable safety profile, as they are associated with a lower risk of toxicity and fewer adverse effects. This is particularly crucial for chronic conditions such as MSDs, which are frequently treated with long-term medication (Johnson and Cosgrove, 2015). Furthermore, herbal medicines are frequently perceived as natural and less invasive by patients, which influences their acceptance and compliance with treatment regimens (Karimi et al., 2015). Inflammation plays a central role in the pathogenesis of MSDs, contributing to pain, swelling, and tissue damage. Numerous plant-derived compounds exhibit potent anti-inflammatory properties by inhibiting pro-inflammatory mediators, such as cytokines, prostaglandins, and leukotrienes. Examples include curcumin from turmeric, boswellic acids from frankincense, and flavonoids from ginger, which have demonstrated efficacy in alleviating symptoms of arthritis and other inflammatory conditions (Nunes et al., 2020). Phytochemicals such as alkaloids, saponins, and capsaicin act on pain receptors, modulate neurotransmitter release and enhance endogenous pain control mechanisms. Traditional analgesic herbs like willow bark have inspired the development of aspirin, a widely used pain reliever for musculoskeletal pain (Kumar et al., 2023). Certain novel synergistic interactions among various phytochemicals essential for optimizing treatment results have been reported. A notable example is the synergistic action of curcumin and piperine in augmenting bioavailability and therapeutic efficacy (Boonrueng et al., 2022). The immunomodulatory and adaptogenic properties of plant-derived compounds have been demonstrated to improve overall well-being and resilience, while simultaneously reducing inflammation and pain associated with MSDs. Phytomedicine is becoming more popular among both healthcare professionals and patients as more scientific evidence shows that it can help treat MSD and is safe to use (Di Sotto et al., 2020).
Therefore, phytomedicine is a promising therapeutic option for MSDs due to its historical use, multi-targeted action, safety profile, anti-inflammatory and analgesic effects, chondroprotective properties, and adaptogenic and immunomodulatory effects. Table 3 provides an overview of selected plants used in phytomedicine, their scientific names, families, key components, and therapeutic properties. The medicinal plants were selected based on a combination of traditional use in treating muscle-related ailments and recent scientific studies that suggest their potential therapeutic effects on MDs. The medicinal plant selection criteria that were implemented in this article included the availability of phytochemical data, evidence of efficacy in pre-clinical models, and relevance to the pathophysiology of MDs. This information enhances understanding of the diverse plant-based remedies used in treating MSDs.
The comprehensive table of medicinal plants includes scientific names, families, key components, and therapeutic properties to treat various MSDs.
Plant name | Scientific name | Family | Key components | Therapeutic properties |
---|---|---|---|---|
Coffee | Coffea arabica | Rubiaceae | Caffeine, dimethylxanthines, paraxanthine, theobromine, theophylline, polyphenols | Anti-inflammatory, antioxidant, immunomodulatory. Reduces levels of IL-1a, IL-6, and TNF-α. Enhances nucleic acid synthesis and satellite cell activation. |
Ginger | Zingiber officinale | Zingiberaceae | Gingerol, shogaol, other chemical moieties | Antioxidant, anti-inflammatory. Inhibits prostaglandin and leukotriene biosynthesis, and the synthesis of pro-inflammatory cytokines IL-1, IL-8, and TNF-α. Effective in alleviating muscle discomfort and inflammation. |
Turmeric | Curcuma longa | Zingiberaceae | Curcumin | Antioxidant, anti-inflammatory, antibacterial, anticancer. Reduces NF-кB signaling, proteolysis, and muscle inflammation. Promotes muscle regeneration after injury and reduces inflammation from exercise-induced muscle damage. |
Aloe vera | Aloe barbadensis | Liliaceae | Anthraquinones, fatty acids, flavonoids, lectins, terpenoids, polysaccharides, vitamins, minerals | Anti-inflammatory, antioxidant. Inhibits prostaglandin-E2 and inducible nitric oxide. Useful in treating allergic reactions, arthritis, and inflammatory conditions related to MSDs. |
Lemon | Citrus limon | Rutaceae | Flavones, flavanones, flavanols, essential oils | Antioxidant, anti-inflammatory. Scavenges free radicals, reduces ROS, prostaglandins, and alleviates joint inflammation and arthritis. |
Liquorice | Glycyrrhiza glabra | Leguminosae | Flavonoids, triterpenes, saponins, polysaccharides, glycosides | Antioxidant, anti-inflammatory. Inhibits production of IL-6, NO, PGE2, and TNF-α. Exhibits estrogen-like activity beneficial for skeletal muscles. |
Bitter orange | Citrus aurantium | Rutaceae | Flavonoids (hesperidin, nobiletin, naringin), essential oils | Anti-inflammatory, antioxidant. Inhibits iNOS, COX-2, IL-6, and TNF-α. Reduces muscle inflammation and proteolysis. |
Saptaparni | Alstonia scholaris | Apocynaceae | Indole alkaloids, iridoids, monoterpenoids, terpenoids, echitamine, tubotaiwine | Anti-inflammatory, analgesic. Reduces leukocyte migration and levels of COX, LOX, PGE2, and NO. Effective in pain and inflammation models. |
Sweet potato | Ipomoea batatas | Convolvulaceae | Rutin, gallic acid, quercetin, kaempferol, vitamins, polyphenols, phenolic compounds, triterpenes | Anti-inflammatory, antioxidant. Suppresses pro-inflammatory cytokines PGE2, IL-1β, and TNF-α. Reduces NO levels and is effective in ameliorating inflammation. |
Chirayita | Swertia chirayita | Gentianaceae | Xanthone derivatives, antioxidants | Anti-inflammatory, analgesic. Reduces pro-inflammatory cytokines TNF-α and IL-1α. Exhibits significant reduction in edema and pain in inflammation models. |
Strychnine tree | Strychnos nux-vomica | Loganiaceae | Coumarins, kaempferol-3-rutinoside, kaempferol-7-glucoside, quercetin-3-rhamnoside, rutin | Anti-inflammatory, antipyretic, analgesic. Reduces PGE2 and TNF-α levels. Demonstrates anti-arthritic activities and reduces vascular permeability. |
Rosemary | Rosmarinus officinalis | Lamiaceae | α-Pinene, caffeic acid, camphor, carnosic acid, carnosol, chlorogenic acid, eucalyptol | Antioxidant, anti-inflammatory, antimicrobial, antiproliferative. Effective in reducing inflammation and protecting against oxidative stress. |
Palo azul | Eysenhardtia polystachya | Fabaceae | Chalcones, coumarins, flavonoids, flavones, isoflavonoids | Anti-inflammatory. Inhibits cytokines PGE2, IL-1β, TNF-α, and LTB4. Reduces levels of lipoxygenase and xanthine-oxidase. Effective in treating rheumatoid arthritis and associated inflammation. |
Cinnamomum porrectum | Cinnamomum porrectum (Roxb.) Kosterm. | Lauraceae | - | Anti-inflammatory, analgesic. Used for muscle pain, leg/thigh symptom/complaint, and flank/axilla symptom/complaint. |
Clinacanthus nutans | Clinacanthus nutans (Burm. f.) Lindau | Acanthaceae | - | Anti-inflammatory, analgesic. Used for muscle pain. |
Cratoxylum formosum | Cratoxylum formosum Dyer | Hypericaceae | - | Anti-inflammatory, analgesic. Used for muscle pain. |
Croton caudatus | Croton caudatus Geiseler | Euphorbiaceae | - | Anti-inflammatory, analgesic. Used for muscle pain. |
Curculigo latifolia | Curculigo latifolia Dryand. ex W.T. Aiton | Hypoxidaceae | - | Anti-inflammatory, analgesic. Used for muscle pain. |
Desmodium styracifolium | Desmodium styracifolium (Osb.) Merr. | Leguminosae | - | Anti-inflammatory, analgesic. Used for muscle pain. |
Dioscorea hispida | Dioscorea hispida Dennst. | Dioscoreaceae | - | Anti-inflammatory, analgesic. Used for muscle pain. |
Drymaria diandra | Drymaria diandra Blume | Caryophyllaceae | - | Anti-inflammatory, analgesic. Used for muscle pain. |
Emilia sonchifolia | Emilia sonchifolia (L.) DC. ex Wight | Asteraceae | - | Anti-inflammatory, analgesic. Used for back pain, leg/thigh symptom/complaint, and muscle pain. |
Euodia meliaefolia | Euodia meliaefolia Benth. | Rutaceae | - | Anti-inflammatory, analgesic. Used for back pain and flank/axilla symptom/complaint. |
Eurycoma longifolia | Eurycoma longifolia Jack | Simaroubaceae | - | Anti-inflammatory, analgesic. Used for muscle pain. |
Ficus fistulosa | Ficus fistulosa Reinw. ex Blume | Moraceae | - | Anti-inflammatory, analgesic. Used for muscle pain. |
Gynura procumbens | Gynura procumbens (Lour.) Merr. | Asteraceae | - | Anti-inflammatory, analgesic. Used for back pain, leg/thigh symptom/complaint, and muscle pain. |
Heliotropium indicum | Heliotropium indicum L. | Boraginaceae | - | Anti-inflammatory, analgesic. Used for muscle pain. |
Hibiscus sabdariffa | Hibiscus sabdariffa L. | Malvaceae | - | Anti-inflammatory, analgesic. Used for back pain, leg/thigh symptom/complaint, and muscle pain. |
Holarrhena pubescens | Holarrhena pubescens Buch.-Ham. | Apocynaceae | - | Anti-inflammatory, analgesic. Used for back pain and flank/axilla symptom/complaint. |
Hyptis suaveolens | Hyptis suaveolens (L.) Poit. | Lamiaceae | - | Anti-inflammatory, analgesic. Used for muscle pain. |
Jasminum adenophyllum | Jasminum adenophyllum Wall. | Oleaceae | - | Anti-inflammatory, analgesic. Used for muscle pain. |
Jasminum auriculatum | Jasminum auriculatum Vahl | Oleaceae | - | Anti-inflammatory, analgesic. Used for muscle pain. |
Justica adhatoda | Justica adhatoda L. | Acanthaceae | - | Anti-inflammatory, analgesic. Used for muscle pain. |
Leea indica | Leea indica (Burm. f.) Merr. | Vitaceae | - | Anti-inflammatory, analgesic. Used for muscle pain. |
Limnophila rugosa | Limnophila rugosa (Roth) Merr. | Plantaginaceae | - | Anti-inflammatory, analgesic. Used for muscle pain. |
Litsea glutinosa | Litsea glutinosa (Lour.) C.B. Rob. | Lauraceae | - | Anti-inflammatory, analgesic. Used for back pain and leg/thigh symptom/complaint. |
Lonicera japonica | Lonicera japonica Thunb. | Caprifoliaceae | - | Anti-inflammatory, analgesic. Used for muscle pain. |
Macaranga denticulata | Macaranga denticulata (Blume) Muell. Arg. | Euphorbiaceae | - | Anti-inflammatory, analgesic. Used for muscle pain. |
Mammea siamensis | Mammea siamensis (Miq.) T. Anderson | Clusiaceae | - | Anti-inflammatory, analgesic. Used for muscle pain. |
Mangifera indica | Mangifera indica L. | Anacardiaceae | - | Anti-inflammatory, analgesic. Used for back pain. |
Markhamia stipulata | Markhamia stipulata (Wall.) Seem. ex K. Schum. | Bignoniaceae | - | Anti-inflammatory, analgesic. Used for muscle pain. |
Momordica charantia | Momordica charantia L. | Cucurbitaceae | - | Anti-inflammatory, analgesic. Used for flank/axilla symptom/complaint. |
Morinda coreia | Morinda coreia Buch.-Ham. | Rubiaceae | - | Anti-inflammatory, analgesic. Used for back pain, flank/axilla symptom/complaint, leg/thigh symptom/complaint, and muscle pain. |
Morinda elliptica | Morinda elliptica (Hook. f.) Ridl. | Rubiaceae | - | Anti-inflammatory, analgesic. Used for muscle pain. |
Musa sapientum | Musa sapientum L. | Musaceae | - | Anti-inflammatory, analgesic. Used for back pain. |
Naravelia laurifolia | Naravelia laurifolia Wall. | Ranunculaceae | - | Anti-inflammatory, analgesic. Used for muscle pain. |
Oroxylum indicum | Oroxylum indicum (L.) Kurz | Bignoniaceae | - | Anti-inflammatory, analgesic. Used for muscle pain. |
Paederia foetida | Paederia foetida L. | Rubiaceae | - | Anti-inflammatory, analgesic. Used for muscle pain. |
Phyllanthus amarus | Phyllanthus amarus Schumach. & Thonn. | Euphorbiaceae | - | Anti-inflammatory, analgesic. Used for muscle pain. |
Piper betle | Piper betle L. | Piperaceae | - | Anti-inflammatory, analgesic. Used for muscle pain. |
Polygonum chinense | Polygonum chinense L. | Polygonaceae | - | Anti-inflammatory, analgesic. Used for muscle pain. |
Senna alata | Senna alata (L.) Roxb. | Leguminosae | - | Anti-inflammatory, analgesic. Used for muscle pain. |
Siphonodon celastrineus | Siphonodon celastrineus Griff. | Celastraceae | - | Anti-inflammatory, analgesic. Used for muscle pain. |
Solanum procumbens | Solanum procumbens Lour. | Solanaceae | - | Anti-inflammatory, analgesic. Used for muscle pain. |
Spilanthes acmella | Spilanthes acmella (L.) L. | Asteraceae | - | Anti-inflammatory, analgesic. Used for muscle pain. |
Streblus asper | Streblus asper Lour. | Moraceae | - | Anti-inflammatory, analgesic. Used for muscle pain. |
Syzygium grande | Syzygium grande (Wight) Walp. | Myrtaceae | - | Anti-inflammatory, analgesic. Used for muscle pain. |
Tabernaemontana divaricata | Tabernaemontana divaricata (L.) R. Br. ex Roem. & Schult. | Apocynaceae | - | Anti-inflammatory, analgesic. Used for muscle pain. |
Terminalia chebula | Terminalia chebula Retz. | Combretaceae | - | Anti-inflammatory, analgesic. Used for muscle pain. |
Vitex trifolia | Vitex trifolia L. | Verbenaceae | - | Anti-inflammatory, analgesic. Used for muscle pain. |
Wrightia pubescens | Wrightia pubescens R. Br. | Apocynaceae | - | Anti-inflammatory, analgesic. Used for muscle pain. |
Zanthoxylum limonella | Zanthoxylum limonella (Dennst.) Alston | Rutaceae | - | Anti-inflammatory, analgesic. Used for muscle pain. |
Zingiber montanum | Zingiber montanum (J.Koenig) Link ex A. Dietr. | Zingiberaceae | - | Anti-inflammatory, analgesic. Used for back pain, flank/axilla symptom/complaint, leg/thigh symptom/complaint, muscle pain, and sprain/strain of joint NOS. |
Zingiber zerumbet | Zingiber zerumbet (L.) Roscoe ex Sm. | Zingiberaceae | - | Anti-inflammatory, analgesic. Used for back pain, flank/axilla symptom/complaint, leg/thigh symptom/complaint, muscle pain, and sprain/strain of joint NOS. |
Abbreviations: IL, interleukin; MSD, musculoskeletal disorders; ROS, reactive oxygen species; TNF, tumor necrosis factor-α.
Clinical trials and research studies on medicinal plants for MD
Recent studies have demonstrated the potential of medicinal plants to treat MD. Epigallocatechin gallate (EGCG), a green tea (Camellia sinensis) polyphenol, was the subject of one study conducted by Dorchies et al. (2006). After administering green tea extract to the mice, the histopathology and muscle function were assessed. The results demonstrated that EGCG treatment, which has antioxidant properties, improved muscle function and decreased muscle degeneration. The main mechanism of action of EGCG was found to be its capacity to reduce ROS and modulate inflammatory cytokines. EGCG was found to enhance cell membrane stability in dystrophic muscles by upregulating utrophin expression, a protein that compensates for dystrophin absence. EGCG also modulates calcium homeostasis by reducing calcium channel activity, which is often dysregulated in MD, preventing calcium overload in muscle cells and reducing cellular damage. It also has potent anti-inflammatory effects by inhibiting the NF-κB pathway, which is often upregulated in MD, reducing pro-inflammatory cytokines, reducing inflammation and muscle degeneration. This research emphasizes the potential of green tea polyphenols to manage MD and to treat other conditions (Dorchies et al., 2006). In turmeric (Curcuma longa), researchers have discovered that curcumin, a polyphenolic compound found in turmeric, can effectively manage degenerative and inflammatory diseases. In addition to improvements in muscle strength and function, a study conducted on mdx mice revealed significant reductions in muscle inflammation and fibrosis. These effects were attributed to curcumin’s anti-inflammatory and antioxidant characteristics, particularly its capacity to block NF-κB signaling. Curcumin, an antioxidant, acts by neutralizing ROS and reactive nitrogen species in muscle cells, protecting them from oxidative damage and improving muscle function. It also inhibits the TGF-β signaling pathway, which is involved in fibrosis, preventing muscle fibrosis. Curcumin also modulates inflammatory pathways, inhibiting enzymes involved in the inflammatory process, leading to reduced inflammation and improved muscle health (Ceco and McNally, 2013). Another study revealed the rejuvenating effects of ashwagandha, an adaptogenic herb used in Ayurvedic medicine, investigated in mdx mice. According to the study, the extract enhanced muscle strength and decreased oxidative damage, which may indicate that it has a preventive effect on muscle deterioration. Some of the primary mechanisms of this effect were its adaptogenic and anti-inflammatory properties, as well as its capacity to improve mitochondrial function. Withaferin A from ashwagandha (Withania somnifera) improves protein homeostasis by enhancing the expression of heat shock proteins, which are essential for protein folding and protection against stress-induced damage in muscle cells. It also enhances mitochondrial function by increasing biogenesis and efficiency, leading to better energy production and reduced muscle fatigue. Similar to curcumin, withaferin A inhibits NF-κB and other pro-inflammatory signaling pathways, reducing inflammation and promoting muscle repair (Mikulska et al., 2023). Similarly, Kim et al. (2017) examined the potential advantages of Panax ginseng in muscle regeneration in a separate study. They gave ginseng extract to mdx mice and measured inflammation and muscle regeneration. The extract was found to enhance muscle histology, decrease inflammation, and enhance the expression of genes specific to muscles. It was discovered that the active ingredient, ginsenosides, improved muscle repair processes. Ginsenosides promote muscle regeneration by stimulating satellite cell activation and differentiation, crucial for muscle repair after injury. They also have anti-apoptotic effects by modulating Bcl-2 family proteins, preventing apoptosis, and preserving muscle cell integrity. Ginsenosides also have anti-inflammatory effects by inhibiting inflammatory mediators like tumor necrosis factor (TNF)-α and interleukin (IL)-6, reducing chronic inflammation and muscle damage associated with MD (Kim et al., 2017). Resveratrol, a polyphenol found in grapes and berries, has been found to have anti-inflammatory and antioxidant properties. A study by Gordon et al. (2014) revealed that resveratrol treatment improved muscle function and reduced oxidative stress and inflammation markers in mdx mice. This was due to resveratrol’s antioxidant activity and its ability to modulate cellular signaling pathways involved in muscle repair. Resveratrol activates SIRT1, a sirtuin involved in cellular stress resistance and mitochondrial biogenesis, leading to improved mitochondrial function and energy metabolism in muscle cells. As an antioxidant, it reduces oxidative stress by scavenging free radicals and enhancing the expression of antioxidant enzymes like superoxide dismutase and catalase. Resveratrol also modulates inflammatory pathways by inhibiting NF-κB and reducing pro-inflammatory cytokines, reducing muscle inflammation and promoting muscle repair (Gordon et al., 2014). Studies suggest that medicinal plants and their compounds have potential as therapeutic agents for MDs, offering benefits like reduced inflammation, oxidative stress reduction, and improved muscle function. These compounds can improve membrane stability, calcium homeostasis, inflammation, and oxidative stress, making them a promising complementary approach to traditional MD therapies. Further research, including clinical trials in humans, is needed to establish their efficacy and safety, enabling their integration into conventional MD treatment regimens.
Certain limitations in medicinal plant use for MD
The use of medicinal plants in treating MD presents both opportunities and challenges. Addressing these challenges is crucial for effectively translating plant-based therapies into clinical practice. Variability in compound concentration, potential side effects, standardization issues, and regulatory hurdles are some of the main challenges. Geographical and environmental factors, such as soil quality, climate, and altitude, significantly influence the concentration of bioactive compounds in medicinal plants (Christensen, 2009). As an example, the concentration of ginsenosides in P. ginseng may vary considerably depending on whether it is cultivated in North America, China, or Korea. This variability makes it difficult to produce uniform plant extracts with consistent therapeutic properties (Chen et al., 2019). Moreover, different parts of a plant, such as roots, leaves, and flowers, often contain different levels of active compounds. For example, the curcuminoid content in C. longa is primarily concentrated in the rhizomes, whereas the leaves and stems contain significantly lower concentrations (Fuloria et al., 2022). This variability necessitates precise identification and use of specific plant parts to achieve the desired therapeutic effect. Therefore, addressing these challenges is essential for the successful translation of plant-based therapies into clinical practice. The timing and methods of harvesting and processing of medicinal plants significantly impact their potency and stability. Improper harvesting or improper drying and storage can lead to degradation or loss of bioactive ingredients. Optimal harvesting and processing conditions are crucial for maintaining the efficacy of medicinal plant products (Klein-Junior et al., 2021). Despite being considered safer alternatives, medicinal plants can cause adverse effects and interact with conventional medications. Some plants, like green tea polyphenols (EGCG), can cause hepatotoxicity or allergic reactions if consumed in large amounts or without proper knowledge. These adverse effects emphasize the need for careful dosage and monitoring (Galati and O’Brien, 2004). Medicinal plants can interact with pharmaceuticals, altering their effectiveness or increasing the risk of side effects. St. John’s wort (Hypericum perforatum), for example, is known to interact with numerous medications, including anticoagulants and antidepressants, by inducing cytochrome P450 enzymes. Understanding these interactions is crucial when combining plant-based therapies with conventional treatments (Izzo and Ernst, 2001, 2009). Standardization is essential for ensuring the safety and efficacy of medicinal plant products, but achieving it can be challenging due to the lack of standardized protocols for cultivation, extraction, and formulation. Establishing standardized procedures is crucial for producing reliable and effective herbal medicines (Muyumba et al., 2021). The quality control of medicinal plant extracts is crucial for their purity and potency, but inconsistent practices across the industry can lead to variable product quality. Regulatory hurdles include the complex landscape of medicinal plants, which are often classified as dietary supplements rather than pharmaceuticals, resulting in less stringent oversight. This can lead to variability in product quality and safety (Kibwage et al., 2005). Clinical trials for medicinal plants are complex due to their composition and variability, and regulatory agencies like the food and drug administration European medicines agency require extensive safety and efficacy data (Ekor, 2014). Addressing these challenges is essential for gaining regulatory approval and integrating medicinal plants into mainstream medical practice (Bent, 2008). Lastly, securing market authorization for medicinal plant products requires navigating a complex regulatory environment and demonstrating consistent manufacturing practices, safety, and therapeutic efficacy. Overcoming these hurdles is crucial for the widespread acceptance and clinical use of medicinal plant-based therapies (Heinrich, 2015). The integration of medicinal plants into mainstream medical practice is critical to ensuring their safety, efficacy, and reliability. Addressing variability and standardization issues will lead to consistent therapeutic outcomes. Regulatory compliance is necessary for medicinal plants to be accepted and utilized in clinical settings. Understanding and addressing these challenges allows healthcare providers to provide a more holistic and integrative approach to MD treatment, potentially improving patient outcomes. This holistic treatment approach has the potential to improve patient outcomes and provide more effective treatment for a variety of medical conditions.
Challenges and future directions
The examination of medicinal plants as therapeutic agents for MD is still in its infancy, and there are numerous gaps in current knowledge that require resolution. Comprehensive studies are required to elucidate these pathways and identify important molecular targets, as the molecular mechanisms through which these compounds affect muscle cells are not entirely understood (Sofowora et al., 2013; Gogoi et al., 2023). Standardization and quality control are also essential to maintain consistency in active compounds as variability can result in uneven therapeutic results (Wang et al., 2023). It is difficult to find clinical evidence regarding the efficacy and safety of medicinal plants in MD patients due to the absence of rigorous clinical trials. Therefore, to provide strong clinical evidence, randomized controlled trials must be carefully planned (Ekor, 2014). Future research directions in biotechnology include genomic and metabolomic approaches to understand the genetic basis of MD and the metabolic pathways influenced by medicinal plants. Synthetic biology can improve the availability and standardization of these therapies by engineering plants or microorganisms to produce higher yields of bioactive compounds (Manickam et al., 2023). Additionally, nanoformulations of plant-derived compounds can enhance their bioavailability, stability, and targeted delivery to muscle tissues, potentially increasing their therapeutic efficacy (Hazarika et al., 2020). Furthermore, the combination therapies could provide new insights into optimizing treatment regimens for MD (Vaou et al., 2022). However, personalized medicine can tailor treatments based on individual genetic and metabolic profiles, improving outcomes (Goetz and Schork, 2018). Integrative care models, which combine conventional and alternative therapies, can enhance patient care and acceptance of medicinal plant-based treatments (Mortada, 2024). Furthermore, the use of CRISPR/Cas9 technology for genome editing may result in plant variants with improved therapeutic properties, allowing for precise modification of genes involved in the biosynthesis of key bioactive compounds (Jaganathan et al., 2018). Metabolic engineering can also improve the production pathways of bioactive compounds in plants, increasing yield while decreasing variability (El-Desoky et al., 2022). Besides, high-throughput screening techniques can speed up the discovery of effective plant-derived compounds by testing large libraries of natural extracts against MD-related targets (Atanasov et al., 2015). Advanced drug delivery systems, such as liposomes and polymeric nanoparticles, can enhance the delivery and efficacy of plant-derived therapies in MD treatment (Patra et al., 2018). Evidence-based integrative approaches that combine medicinal plants with established therapies can provide a comprehensive treatment strategy for multiple aspects of MD pathology (Seetharaman et al., 2021). Increasing healthcare providers’ training and education on medicinal plants and integrative therapies can help them become more widely used in clinical practice (Mishra et al., 2015). Encouraging research and innovation in medicinal plants for MD treatment is critical for meeting unmet needs, improving patient outcomes, promoting holistic care, and raising awareness and acceptance. Current treatments are primarily symptom-focused; however, innovative research can lead to more effective and comprehensive therapies. Integrating medicinal plants into existing treatments can improve patient outcomes and promote a more holistic approach to MD treatment. Increased research and clinical evidence can also help healthcare providers and patients understand and accept medicinal plant-based therapies, promoting their widespread use and integration into standard care practices.
CONCLUSION
MDs are hereditary disorders in which the skeletal muscles undergo progressive degeneration and weaken. However, the current treatment strategies are symptomatic and face immune responses, low efficiency of delivery, and poor long-term effectiveness. Various phytoconstituent molecules derived from medicinal plants, including C. sinensis, C. longa, W. somnifera, P. ginseng and resveratrol, have shown excellent potential in pre-clinical studies for modulating inflammation and oxidative stress with improvement in muscle function. However, challenges like variability in bioactive compound concentration, potential side effects, standardization issues, and regulatory hurdles must be addressed for effective integration into MD treatment regimens. Rigorous clinical trials and standardization protocols are essential for validating the efficacy and safety of these plant-based therapies. Future research should focus on understanding the molecular mechanisms of plant-derived compounds, optimizing their production, and integrating these therapies into personalized treatment strategies.