INTRODUCTION
Overview of sports prostheses for children and the importance of self-hygiene
Sports prostheses have revolutionized the lives of children with limb loss, providing them with the opportunity to engage in various physical activities and sports ( Johannes et al., 2019; Yu et al., 2021). These specialized devices are designed to enhance mobility, restore functionality, and promote an active lifestyle for children who have experienced limb loss due to congenital conditions, accidents, or medical interventions. However, along with the many benefits they offer, sports prostheses also present unique challenges related to hygiene. This review article provides an overview of sports prostheses for children and emphasizes the importance of self-hygiene practices in maintaining their health and well-being. Sports prostheses are custom-made devices that are specifically tailored to meet the unique needs and preferences of each individual child. They are designed to provide stability, support, and a natural range of motion, enabling children to participate in various sports and physical activities with confidence. These prostheses can be categorized into different types based on the level of amputation, including below-knee (transtibial), above-knee (transfemoral), and upper-limb prostheses. Advanced technologies, such as microprocessor-controlled knees and myoelectric hands, have significantly improved the functionality and comfort of these devices, enhancing the overall experience for young athletes ( Moore et al., 1989; Nielsen et al., 1989).
Maintaining proper self-hygiene practices is crucial for children using sports prostheses. Several factors contribute to the significance of self-hygiene in this context. Prolonged use of sports prostheses increases the risk of microbial colonization and infection. Sweat, heat, and friction created during physical activities create an environment conducive to bacterial growth. Adequate self-hygiene practices, including regular cleaning and disinfection, can help prevent the accumulation of bacteria and minimize the risk of infections. The junction where the remaining limb connects with the prosthesis is susceptible to skin irritations and abrasions. When children uphold proper hygiene, it can diminish the chances of skin damage, pressure ulcers, and the discomfort stemming from friction. Maintaining cleanliness additionally supports the optimal health of the skin and enhances the overall comfort and fit of the prosthesis. Accumulated sweat and bacteria can lead to unpleasant odors emanating from sports prostheses. This can cause social discomfort and self-consciousness for children. By adopting proper hygiene practices, such as regular washing and airing out of the prostheses, children can effectively manage odor and boost their self-confidence. Sports prostheses are valuable investments, both in terms of cost and functionality. Practicing good self-hygiene habits helps prolong the lifespan of the prosthetic devices by minimizing wear and tear, preventing degradation of materials, and reducing the need for frequent repairs or replacements. To ensure effective self-hygiene, children using sports prostheses should be educated and encouraged to adopt the following practices. Children should be instructed on how to clean their prostheses thoroughly, paying attention to all components, including sockets, liners, and suspension systems. Mild soap and water can be used for routine cleaning, while specific cleaning agents may be recommended for disinfection. After cleaning, it is essential to allow the prostheses to dry completely before wearing them again. Moisture can encourage bacterial growth and cause skin irritations. Providing proper ventilation and airing out the prostheses overnight can help maintain dryness and freshness. Children should be educated on proper skin care routines, including moisturizing the residual limb to prevent dryness and maintaining skin integrity.
Introduction to the concept of antibacterial silver nanoparticles and their potential application
For close to a decade, scientists have engaged in discussions regarding the mechanisms through which silver nanoparticles (AgNPs) exert their toxicity against bacteria and other organisms. In recent years, the field of nanotechnology has emerged as a promising area with a wide range of applications across various sectors, including healthcare. Historically, silver in its various forms has been employed as an antimicrobial agent, either independently or in conjunction with other technologies. The bactericidal effects of silver-holding materials have been frequently examined based on their silver ion substance. One such application is the use of antibacterial AgNPs to combat microbial contamination and prevent infections. Silver (Ag) has shown significant antibacterial efficacy and has been extensively utilized in medicine ( Brennan et al., 2015; Mahfooz et al., 2018; Sarraf et al., 2018). Furthermore, Ag can be transformed into AgNPs using nanotechnology, resulting in enhanced physical, chemical, and biological characteristics. AgNPs possess unique properties that make them highly effective against a broad spectrum of pathogens, including bacteria, viruses, and fungi ( Razzaq et al., 2021; Khalid et al., 2021a, b, 2022; Ahmad et al., 2022; Irshad et al., 2022; Habib et al., 2023). Figure 1 shows the approaches and primary methods for incorporating AgNPs onto the surface of implants. AgNPs are defined as a nanomaterial with all its dimensions in the range of 1–100 nm. These have shown greater capacity and higher surface (area-to-volume ratio) compared to silver in its bulk form. The high surface-to-volume ratio of AgNPs enhances their reactivity and allows for efficient interactions with biological entities. AgNPs have been extensively studied for their antibacterial properties due to their ability to inhibit microbial growth and disrupt vital cellular processes. These nanoparticles can penetrate bacterial cell membranes, leading to structural damage, DNA disruption, and cell death.
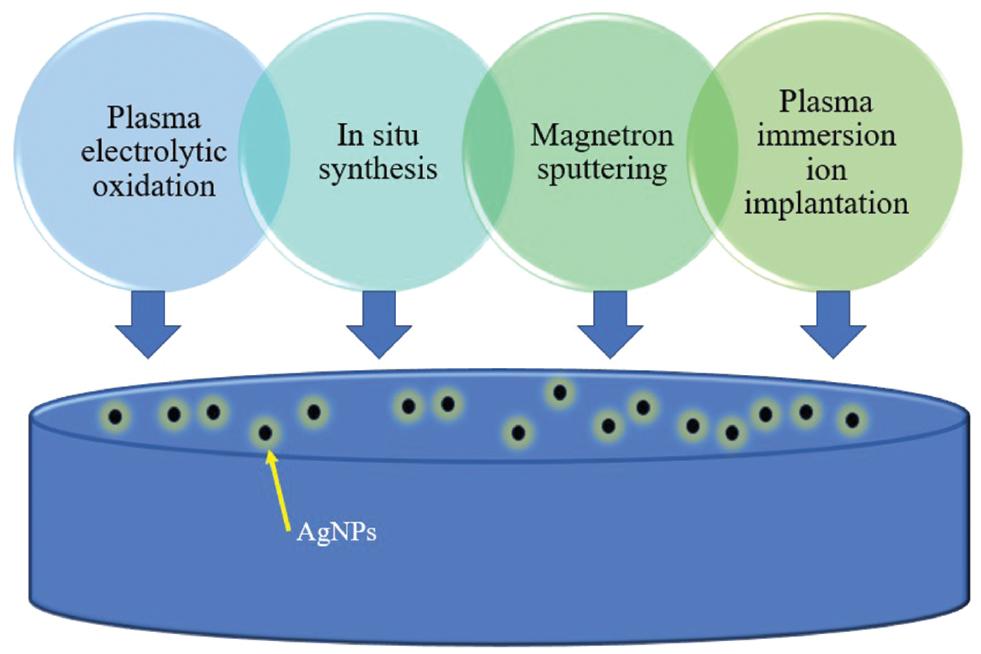
Approaches and primary methods for incorporating AgNPs onto the surface of implants. Abbreviation: AgNPs, silver nanoparticles.
The antimicrobial mechanism of AgNPs involves several key factors. First, silver ions are released from the nanoparticles, which possess strong affinity to bacterial cell components such as proteins, enzymes, and DNA. This interaction disrupts the normal functioning of these essential components, impairing bacterial viability. Second, AgNPs induce the generation of reactive oxygen species (ROS) within bacterial cells, causing oxidative stress and further damaging cellular structures. Additionally, they exhibit a size-dependent effect, as smaller nanoparticles have been found to have higher antimicrobial activity due to their increased surface area and higher ion release. The potential applications in healthcare are vast and hold great promise for addressing numerous challenges. In the context of self-hygiene of sports prostheses for children, it can play a crucial role in preventing microbial colonization and reducing the risk of infections ( Capellato et al., 2020; Ghilini et al., 2021). Incorporating AgNPs into the design and manufacturing of prosthetic materials, such as silicone liners or socket interfaces, can provide a self-sterilizing surface that hinders bacterial growth and promotes a hygienic environment. This can significantly improve the overall hygiene practices and well-being of children utilizing sports prostheses. Furthermore, it has shown efficacy in wound healing applications ( Sohi et al., 2021). They possess unique properties that promote tissue regeneration, reduce inflammation, and prevent bacterial infections in wounds ( Sohi et al., 2021). AgNP-based dressings and coatings have been developed to enhance the healing process and minimize the risk of complications associated with wound infections. Their broad-spectrum antibacterial activity makes them particularly valuable in managing complex and drug-resistant infections. Beyond healthcare, AgNPs find applications in various other sectors. They are utilized in textiles, such as sportswear and socks, to impart antimicrobial properties and reduce odor-causing bacteria. AgNPs are also incorporated into consumer products like cosmetics, soaps, and toothpaste for their antibacterial effects. Additionally, they hold promise in water purification systems, where they can efficiently eliminate microbial contaminants and provide safe drinking water. While they offer significant potential in various applications, it is crucial to address concerns regarding their safety and potential toxicity. Research is ongoing to understand the environmental impact and long-term effects of AgNPs ( Brennan et al., 2015; Cui et al., 2018; Mahfooz et al., 2018; Rafieerad et al., 2019; An et al., 2020; Czechowska et al., 2021; Pangli et al., 2021; Poon et al., 2021; Sakthi Devi et al., 2022; Fathy Abo-Elmahasen et al., 2023). Striking a balance between the antimicrobial benefits and safety considerations is essential for their responsible and effective utilization. Their unique properties and broad-spectrum antimicrobial activity make them valuable in healthcare applications, including the self-hygiene of sports prostheses for children. By incorporating AgNPs into prosthetic materials, a hygienic environment can be promoted, reducing the risk of infections and enhancing the overall well-being ( Akhavan et al., 2018; Sarraf et al., 2018; Vale et al., 2019; Xie et al., 2019; Devaraj et al., 2020; Sohi et al., 2021; Bee et al., 2022; Nathanael et al., 2022; Padmanabhan et al., 2022; Rodriguez Barroso et al., 2023).
HYGIENE CHALLENGES IN SPORTS PROSTHESES FOR CHILDREN
Sports prostheses for children, while offering significant advantages in terms of mobility and functionality, present unique hygiene challenges. Prolonged use and physical activity can contribute to various issues related to hygiene and cleanliness. This section focuses on the identification of common hygiene challenges associated with sports prostheses, including microbial colonization, odor, and skin irritations. Microbial colonization is a significant concern when it comes to sports prostheses. The warm and moist environment created by perspiration and prolonged use of the prosthetic device provides an ideal breeding ground for bacteria, fungi, and other microorganisms. These microorganisms can colonize the prosthetic components, such as the socket, liners, suspension systems, and straps. The presence of bacteria on the prosthesis increases the risk of infections, including skin infections and soft tissue complications. Moreover, microbial colonization can lead to the development of biofilms, which are complex microbial communities embedded within a protective matrix. Biofilms are highly resistant to antibiotics and host immune responses, making them particularly challenging to eradicate. Odor is another hygiene challenge associated with sports prostheses. Accumulated sweat, bacteria, and other microorganisms on the prosthesis can lead to unpleasant and persistent odors. The interaction between sweat and bacteria produces volatile compounds that contribute to the characteristic odor. Odor-related issues can cause social discomfort and self-consciousness for children using sports prostheses, potentially impacting their confidence and overall well-being. The interface between the residual limb and the sports prosthesis is prone to skin irritations. Friction, pressure, and moisture can cause abrasions, blisters, redness, and skin breakdown. Skin irritations not only cause discomfort and pain but can also increase the risk of infections. In severe cases, persistent skin irritations may lead to the development of pressure sores or ulcers, which require medical attention and may limit the child’s ability to use the prosthesis effectively.
ANTIBACTERIAL PROPERTIES OF AgNPs
Antibacterial AgNPs have gained significant attention due to their potent antimicrobial properties against a broad range of pathogens, including bacteria. They exhibit unique physicochemical properties that enable them to disrupt cellular processes and inhibit bacterial growth. This section provides an explanation of the antimicrobial mechanisms of AgNPs, highlighting their ability to target bacterial cells and impede their survival and proliferation ( Putra, 2018; Sobolev et al., 2019; Hu et al., 2020; Du et al., 2022).
Interaction with bacterial cell membranes
AgNPs interact with the outer cell membranes of bacteria, which consist of phospholipid bilayers and various membrane-associated proteins. The positively charged AgNPs are attracted to the negatively charged bacterial cell surfaces. The nanoparticles can penetrate the cell membrane and disrupt its integrity, leading to increased permeability. This disruption compromises the selective transport of molecules across the membrane, resulting in the leakage of essential cellular components, such as ions, metabolites, and proteins. AgNPs can induce the generation of ROS within bacterial cells. These ROS, such as superoxide radicals (O 2 •-) and hydroxyl radicals (OH •), are highly reactive molecules that cause oxidative stress. AgNPs can directly interact with molecular oxygen (O 2) and generate superoxide radicals through a redox reaction. These radicals can subsequently react with other cellular components, including proteins, lipids, and DNA, leading to cellular damage, disruption of vital cellular processes, and ultimately cell death. It can interfere with the normal functioning of essential enzymes within bacterial cells. Enzymes play critical roles in various cellular processes, including metabolism, DNA replication, and protein synthesis ( Weng et al., 2018; Rafieerad et al., 2019; Bakhsheshi-Rad et al., 2020; Guo et al., 2020; Liu et al., 2022). AgNPs can bind to enzymes, affecting their three-dimensional structure and disrupting their catalytic activity. This inhibition of enzymatic activity hinders essential cellular processes, leading to impaired bacterial growth and survival. They can interact with bacterial DNA, which carries the genetic information necessary for bacterial replication and survival. AgNPs can bind to DNA molecules, leading to structural damage, strand breaks, and interference with DNA replication and transcription. This disruption of the genetic material inhibits bacterial proliferation and impairs the ability of bacteria to adapt to their environment. One of the key mechanisms contributing to the antimicrobial activity of AgNPs is the release of silver ions (Ag +). Upon contact with bacterial cells, it can release Ag + ions, which exhibit potent antimicrobial effects ( Prokopovich et al., 2013; Capellato et al., 2020; Piras et al., 2020). The released Ag + ions can interact with various cellular components, including proteins, enzymes, and DNA. These interactions disrupt essential cellular processes, leading to bacterial cell death.
The combined action of these antimicrobial mechanisms contributes to the efficacy of AgNPs in inhibiting bacterial growth and combating microbial infections. The disruption of bacterial cell membranes, generation of ROS, inhibition of enzymatic activity, interaction with DNA, and silver ion release collectively contribute to the broad-spectrum antimicrobial activity of AgNPs against bacteria. Figure 2 shows the four primary pathways by which AgNPs exert antimicrobial effects. It is important to note that the antimicrobial mechanisms may vary depending on factors such as the size, shape, surface charge, and concentration of the nanoparticles, as well as the specific bacterial species targeted. Ongoing research aims to further elucidate the precise mechanisms of action and optimize the use of AgNPs as effective antibacterial agents.
STUDIES ON SELF-HYGIENE AND AgNPs IN SPORTS PROSTHESES
The effectiveness of antibacterial AgNPs in improving self-hygiene practices, particularly in the context of sports prostheses for children, has been the subject of several studies. These studies have explored the antimicrobial properties of AgNPs, their incorporation into prosthetic materials, and their impact on reducing microbial colonization, preventing infections, and improving overall hygiene ( Basova et al., 2021; Sakthi Devi et al., 2022). Ye et al. (2022) investigated antimicrobial coatings for orthopedic and dental implants. They developed self-assembled antimicrobial amphiphiles using GL13K, an AMP, and enhanced them with AgNPs. These hybrid nanocoatings showed superior antimicrobial effectiveness against implant-related bacteria compared to single AgNP or AMP coatings. Zhang et al. (2018) developed a novel strategy to create polypeptide multilayer films integrated with capsules for co-delivery of multiple drugs using the layer-by-layer self-assembly technology. This approach allowed for high and controllable loading of multiple drugs, achieving pH-responsive and sustained release. The resulting materials effectively co-delivered proteins, nanoparticles, and other therapeutic agents with precise control and desired release durations. These materials demonstrated excellent killing efficacy against Staphylococcus aureus while promoting osteoblast viability and cell adhesion and proliferation. Liu et al. (2022) focused on the development of porous titanium (Ti) as an advanced implant material. The researchers proposed a new method to create micron-sized porous structures on the surface of commercially-pure Ti using a vacuum wetting process with pure silver (Ag). The resulting porous Ti exhibited excellent bio-corrosion resistance, biocompatibility, mechanical properties, and antibacterial activity. The porous structures contained dispersed AgNPs, with average pore sizes ranging from 0.5 to 5 μm. The research delved into the mechanisms responsible for the creation of porous structures and AgNPs, emphasizing the process of Ag volatilization/sublimation within titanium (Ti). This study paves the way for potential advancements in the fabrication of sophisticated porous Ti materials for use in orthopedic implants and various biomedical applications.
Furthermore, a bioactive calcium carbonate (CaCO 3) coating, incorporated with AgNPs, is applied to a conductive PEEK/graphene nanocomposite (P/G) through electrophoretic deposition. The resulting Ca/Ag@P/G composite demonstrates desirable antibacterial properties against S. aureus and Escherichia coli, improved biocompatibility, and enhanced osteogenesis capability. The inclusion of graphene nanosheets provides a photothermal conversion effect that aids in killing bacteria and tumor cells. This multifunctional composite holds promise for application in infectious and tumorous bone repair ( Du et al., 2022). Santos et al. (2020) investigated the impact of gold nanoparticles (AuNPs) and AgNPs on the properties of calcium aluminate cement (CAC) for bone repair applications. The addition of AuNPs enhances flexural stress, reduces porosity and pore diameter, and improves bioactivity. Injectable pastes with a high solid content demonstrate potential for bone defect applications, offering advantages such as better adaptation to the defect geometry, reduced patient trauma, and lower infection risk. However, the addition of AgNPs has negative effects on the mechanical properties of CAC. Overall, the study highlights the influence of nanotechnology in enhancing the properties of CAC for bone modeling. Karunakaran et al. (2019) studied the synthesis of mesoporous Ag-doped hydroxyapatite (HAp) nanorods using biowaste seashells with antibacterial properties. The nanoparticles obtained exhibit a hexagonal crystalline structure and retain the HAp phase even after silver doping. The morphological analysis shows that pure HAp consists of elongated mesoporous nanorods, while the silver-doped HAp nanoparticles have varying dimensions and aspect ratios. The synthesized nanoparticles exhibit high specific surface areas and pore diameters. Moreover, the antibacterial and cytotoxicity studies demonstrate the efficient antibacterial activity and non-toxic nature of the silver-doped HAp nanorods. The ascorbic acid-assisted microwave synthesis method offers a rapid and promising approach for producing HAp nanoparticles with different dimensions and mesoporous structures, making them suitable for implant applications. Furthermore, Table 1 summarizes some important research studies on AgNPs. Overall, these studies collectively highlight the effectiveness of AgNPs in improving self-hygiene practices associated with sports prostheses. The incorporation of AgNPs into different components of the prosthetic device has demonstrated significant antimicrobial activity, reducing microbial colonization and preventing infections. These findings support the potential of AgNPs in enhancing the overall hygiene and well-being of children utilizing sports prostheses. However, further research is needed to explore the long-term effectiveness, safety, and practical implementation of self-hygiene practices.
Summary of some research studies on AgNPs.
No. | Authors | Investigations | Methodology | Outcome |
---|---|---|---|---|
1 | Alzubaidi et al. (2023) | Explored the utilization of ethanolic flaxseed extract as an effective reducing agent in the biosynthesis of AgNPs. | The morphology and structure of AgNPs were evaluated using SEM and XRD analysis. | The antimicrobial efficacy of AgNPs was examined against Escherichia coli, Klebsiella pneumoniae, and Staphylococcus aureus. |
2 | Bano et al. (2023) | AgNPs were synthesized using secondary metabolites. | The synthesis of AgNPs was confirmed using various analytical techniques including UV-Vis spectroscopy, TEM, FTIR spectroscopy, and high-performance liquid chromatography studies. | The potential role of the synthesized AgNPs against multidrug-resistant biofilm forming meningitis-causing microbes was reported. |
3 | Thomas and Thalla (2023) | AgNPs were synthesized from silver nitrate using an aqueous extract of Myristica fragrans seed shells. | The synthesized nanoparticles were characterized using various techniques including small-angle X-ray scattering, XRD, thermogravimetric analysis, and zeta potential measurement. | Under UV light, the results demonstrated a superior photocatalytic degradation efficiency (>90%) for rhodamine B (zwitterionic). |
4 | Yang et al. (2023) | Novel active food packaging films were developed by incorporating AgNPs onto CS and incorporating them into polyvinyl alcohol. | AgNPs were synthesized using a liquid phase chemical reduction method, employing CS and sodium citrate as a friendly reductant for AgNO 3. | The composite film demonstrated exceptional mechanical properties, including a tensile strength of 49.97 MPa and an elongation at break of 275.90%. |
5 | Singh and Mijakovic (2022) | To investigate whether the composition of the biological corona could impact the antimicrobial effectiveness of green AgNPs. | AgNPs were synthesized within Pseudomonas putida KT2440 and E. coli K12 MG1655, followed by testing their efficacy against pathogenic strains belonging to the respective genera. | The findings suggest that nanoparticles synthesized within microorganisms closely related to the target pathogen may exhibit enhanced effectiveness. |
6 | Begum et al. (2022) | Study presents a convenient one-pot synthetic approach for the controlled-size synthesis of AgNPs. | The characterization of the nanoparticles was conducted using spectroscopic techniques including UV-Vis and FTIR. | The results of this research suggest a potential therapeutic application of AgNPs/TA in combination with antibiotics. |
7 | Xiu et al. (2012) | Absence of any direct particle-specific biological effects or toxicity associated with the synthesized AgNPs. | Evidence that the toxicity exhibited by different sizes of AgNPs (coated with PEG or PVP) accurately aligns with the dose–response pattern. | Study concluded that the morphological properties of AgNPs, which are known to impact antimicrobial activity, primarily influence the release of Ag + ions rather than exerting direct effects. |
8 | Baker et al. (2005) | The antibacterial effectiveness of the nanoparticles was assessed by exposing them to a medium containing E. coli. | Antibacterial tests were conducted both in solution and using Petri dishes. | Nanoparticles with smaller sizes and larger surface-to-volume ratios showed enhanced antibacterial activity. |
9 | Chen and Chiang (2008) | Cotton fibers were modified by grafting a chelating monomer, glycidyl methacrylate–iminodiacetic acid. | The formation of CFGI/silver nanocomposites was confirmed by SEM and energy-dispersive X-ray spectrometry. | The weight of Ag + adsorbed by CFGI directly influenced the size of the Ag nanoparticles formed. |
Abbreviations: Ag, silver; AgNPs, silver nanoparticles; CS, chitosan; FTIR, Fourier transform infrared; SEM, scanning electron microscopy; TEM, transmission electron microscopy; TGA, thermal gravimetric analysis; UV-Vis, UV–visible; XRD, X-ray diffraction.
SAFETY CONSIDERATIONS OF AgNPs IN CHILDREN
While antibacterial AgNPs offer significant potential in various applications, including self-hygiene practices for sports prostheses, it is important to consider the safety concerns associated with their use. This discussion focuses on the potential toxicity of AgNPs and their environmental impact, highlighting the need for responsible utilization and ongoing research. The potential toxicity of AgNPs has been a subject of concern. AgNPs can interact with biological systems, and their small size and high surface area-to-volume ratio contribute to their reactivity and potential toxicity. Studies have shown that AgNPs can induce cytotoxicity, oxidative stress, DNA damage, and inflammatory responses in various cell types. However, the toxicity is dependent on several factors, including their size, shape, surface charge, concentration, and duration of exposure. It is crucial to consider these factors when assessing the potential risks associated with the usage. The routes of exposure to AgNPs include inhalation, dermal contact, and ingestion. Inhaling them in occupational settings, such as during their production or application, raises concerns about respiratory health. Dermal contact can occur when using products or materials containing AgNPs, including sports prostheses. While the intact skin acts as a barrier, prolonged or repeated exposure on compromised or damaged skin may increase the risk of adverse effects. Ingesting them can occur inadvertently, particularly in children, through the transfer of AgNP-coated objects to the mouth. Understanding and minimizing exposure routes are essential for mitigating potential risks. Furthermore, AgNPs, when released into the environment, can pose potential risks to ecosystems. It can enter water bodies, soil, and the atmosphere through various pathways, including wastewater discharge, leaching from consumer products, and disposal of AgNP-containing materials. The potential environmental impact includes their accumulation in aquatic organisms, toxicity to microorganisms and aquatic life, and potential disruption of ecological balance. Efforts are underway to study the fate, behavior, and environmental effects of AgNPs to ensure their responsible use and minimize their impact on ecosystems. To address the safety concerns associated, risk assessment and regulation play critical roles. Regulatory authorities, such as the Environmental Protection Agency and the Food and Drug Administration, are actively involved in evaluating the safety of nanomaterials, including AgNPs. Risk assessment studies aim to determine safe exposure limits, identify potential hazards, and develop guidelines for their use in various applications. It is essential to adhere to established regulations, follow proper safety protocols, and engage in ongoing research to better understand the potential risks and ensure the responsible use. While they offer significant potential in enhancing self-hygiene practices, it is important to consider the potential toxicity and environmental impact associated with their use. Comprehending the factors that impact the toxicity of AgNPs, reducing potential exposure pathways, and conducting thorough risk assessments are indispensable for guaranteeing the safe and conscientious application of these nanoparticles. Continual research endeavors and regulatory initiatives play a pivotal role in advancing our comprehension of safety aspects and fine-tuning the utilization of AgNPs across diverse applications.
SUGGESTIONS FOR INCORPORATING AgNPs INTO THE DESIGN AND MANUFACTURING OF SPORTS PROSTHESES TO OPTIMIZE SELF-HYGIENE PRACTICES
The incorporation of antibacterial AgNPs into the design and manufacturing of sports prostheses can significantly enhance self-hygiene practices and reduce the risk of microbial colonization and infections. Table 2 shows the effect of AgNPs on different types of bacteria. Here are some suggestions for incorporating AgNPs into sports prostheses to optimize self-hygiene practices.
Effect of AgNPs on different types of bacteria.
Bacteria | Effect of AgNPs |
---|---|
Escherichia coli | Strong antibacterial activity ( Chen and Chiang, 2008) |
Staphylococcus aureus | Significant inhibition of bacterial growth ( Bee et al., 2022) |
Klebsiella pneumoniae | Effective suppression of bacterial proliferation ( Ansar et al., 2020) |
Streptococcus pyogenes | Prominent bactericidal effect ( Alzubaidi et al., 2023) |
Pseudomonas aeruginosa | Substantial reduction in bacterial viability ( Sasidharan et al., 2020) |
Salmonella enterica | Potent antimicrobial activity, inhibition of bacterial growth ( Majumder et al., 2022) |
Enterococcus faecalis | Marked bacteriostatic and bactericidal properties ( Pranati et al., 2019) |
Acinetobacter baumannii | Notable antimicrobial effect, leading to bacterial inhibition ( Camargo et al., 2023) |
Abbreviation: AgNPs, silver nanoparticles.
AgNP-coated surfaces: Apply a thin layer on the surfaces of sports prostheses, particularly in areas that come into direct contact with the skin. AgNPs can be coated onto the prosthetic socket, liners, suspension systems, and other relevant components. This coating provides a continuous release of silver ions, inhibiting bacterial growth and reducing the risk of infection.
Integration with antimicrobial materials: Incorporate AgNPs into the composition of antimicrobial materials used in sports prostheses. For example, they can be embedded or dispersed within silicone liners or other polymer-based materials. This integration ensures a sustained release of silver ions, creating an inhospitable environment for bacteria and improving self-hygiene.
Nanocomposite structures: Develop nanocomposite structures by incorporating AgNPs into the matrix of sports prostheses. They can be dispersed within the polymer matrix or incorporated into specific regions of the prosthetic device. This approach ensures a uniform distribution throughout the prosthesis, providing continuous antimicrobial protection.
Controlled release systems: Implement controlled release systems within sports prostheses. This can be achieved by encapsulating within biodegradable or stimuli-responsive microspheres or nanoparticles. These systems can release AgNPs in a controlled manner, prolonging their antimicrobial activity and reducing the need for frequent AgNP application or replacement.
Incorporation of AgNPs in textiles and fabrics: Use in the development of antimicrobial textiles and fabrics integrated into sports prostheses. They can be applied to prosthetic socks, liners, or other fabric components to provide an additional layer of antimicrobial protection, reducing microbial colonization and odors.
Hygiene education and maintenance: Alongside their incorporation, emphasize the importance of hygiene education and maintenance for sports prostheses. Educate users, parents, and caregivers on proper cleaning techniques, regular inspection, and maintenance of the prosthetic device. This includes guidelines for cleaning AgNP-coated surfaces and understanding the limitations and lifespan of AgNP functionality.
Collaboration and innovation: Encourage collaboration between prosthetic manufacturers, materials scientists, biomedical engineers, and nanotechnology experts to foster innovation in AgNP incorporation. Exchange knowledge, share research findings, and work together to develop novel approaches and technologies that optimize self-hygiene practices in sports prostheses.
It is essential to note that implementing in sports prostheses should be done responsibly, taking into consideration safety considerations and regulatory guidelines. Additionally, regular monitoring and evaluation of AgNP effectiveness and long-term performance are necessary to ensure their continued efficacy in improving self-hygiene practices. By incorporating into the design and manufacturing of sports prostheses, we can enhance their antimicrobial properties, minimize the risk of infections, and promote better self-hygiene practices for children utilizing these devices.
FUTURE DIRECTIONS AND RECOMMENDATIONS
While significant progress has been made in understanding the antimicrobial properties and potential applications of antibacterial AgNPs, there are still several research gaps and areas that require further exploration. Identifying these research gaps is crucial for advancing the field and optimizing the use. Despite extensive research on the safety of AgNPs, there is a need for long-term safety assessments, especially regarding chronic exposure. Further studies are required to evaluate the potential cumulative effects on human health and the environment over extended periods. This includes investigating the potential for bioaccumulation, organ-specific toxicity, and chronic inflammatory responses associated. There is a lack of standardized testing methods for evaluating the efficacy and safety of AgNPs. Establishing standardized protocols for assessing antimicrobial activity, cytotoxicity, genotoxicity, and other relevant parameters will facilitate accurate comparisons and enable better interpretation of research findings. Consistent methodologies across studies will enhance the reliability and reproducibility of results. While the antimicrobial mechanisms have been partially elucidated, there is still much to learn about their detailed interactions with bacterial cells. Further investigations are needed to better understand the specific molecular targets and pathways affected by AgNPs, as well as the factors influencing their antimicrobial activity. Exploring the variations in AgNP activity against different bacterial strains and species will contribute to a comprehensive understanding of their mechanisms of action. AgNPs release silver ions (Ag +) as a key antimicrobial mechanism. However, the stability and release kinetics in different environments, such as aqueous solutions, biological fluids, and polymer matrices, remain poorly understood. Further studies should focus on elucidating the factors affecting AgNP stability and Ag + release, including pH, temperature, and the presence of various ligands or biomolecules. The environmental fate, transport, and impact are areas that require further investigation. Understanding the behavior of AgNPs in different environmental matrices, such as water and soil, and their interactions with organisms across various trophic levels will help assess their potential risks and develop appropriate mitigation strategies. Exploring the potential synergistic effects in combination with other antimicrobial agents or therapies is an area of interest. Investigating the interactions between AgNPs and antibiotics, antifungal agents, or alternative antimicrobial compounds can lead to the development of novel therapeutic approaches with enhanced efficacy and reduced resistance. While research has shown promise, there is a need for more practical applications and translation of this technology into real-world settings. Further research should focus on developing AgNP-based products, coatings, and materials that can be effectively integrated into healthcare, consumer, and industrial sectors. By addressing these research gaps and exploring these areas, we can enhance our understanding, optimize their applications, and ensure their safe and effective use in various fields, including self-hygiene practices for sports prostheses. Continued research and collaboration among multidisciplinary teams will contribute to the advancement of AgNP technology and its positive impact on human health and the environment.
SUMMARY
Sports prostheses are instrumental in improving the mobility and physical activity levels of children who have experienced limb loss or have limb deficiencies. Nevertheless, these prosthetic tools may bring about issues related to personal hygiene, such as microbial growth, unpleasant odors, and skin irritations. The incorporation of antibacterial AgNPs shows promise in tackling these issues and enhancing the overall quality of life for children who rely on sports prostheses. They possess potent antimicrobial properties that can help combat bacterial colonization on sports prostheses. By incorporating AgNPs into the design and manufacturing of prosthetic components, such as sockets, liners, or suspension systems, the risk of microbial growth can be significantly reduced. This can contribute to maintaining a clean and hygienic environment for the child, minimizing the chances of infections or skin-related complications. Microbial colonization on sports prostheses can lead to unpleasant odors, causing discomfort and social challenges for children. Their antimicrobial properties can effectively inhibit the growth of odor-causing bacteria, thus reducing or eliminating the odor associated with prosthetic devices. This can enhance the child’s confidence, social interactions, and overall well-being. Skin irritations, such as rashes or dermatitis, can occur due to prolonged contact with bacteria on sports prostheses. The ability of AgNPs to inhibit bacterial growth can help prevent such skin irritations, ensuring that the child’s skin remains healthy and free from discomfort. By minimizing skin-related issues, they contribute to improving the child’s comfort, mobility, and quality of life. AgNPs incorporated into sports prostheses can act as a proactive defense against bacterial colonization. By reducing microbial growth, they can minimize the need for frequent and aggressive cleaning, making self-hygiene practices more manageable for children. This simplification of hygiene routines can promote independence, self-confidence, and a sense of empowerment for the child. AgNPs provide a sustained release of silver ions, ensuring continuous antimicrobial protection on sports prostheses. This long-lasting effect reduces the risk of bacterial colonization and infections over extended periods. As a result, children can engage in physical activities and sports with confidence, knowing that their prostheses are safeguarded against microbial threats.
In summary, addressing self-hygiene challenges in sports prostheses for children goes beyond just their antibacterial properties. It involves improving hygiene, reducing odor, and preventing skin irritations, all of which enhance the well-being of children with limb loss or limb deficiencies. These improvements enable greater comfort, social integration, and participation in sports and physical activities, empowering these children. Ongoing research and innovation offer potential for further advancements in self-hygiene practices and overall quality of life for prosthetic users. This review aims to comprehensively explore the role of antibacterial AgNPs in promoting self-hygiene among these children, contributing to existing knowledge and guiding future research in this important field.