Abbreviations:
2D, Two dimensional; 4D Flow, Four-dimensional flow; AR, Aortic regurgitation; ARVC, Arrhythmogenic right ventricular cardiomyopathy; AUC, Area under the curve; CAD, Coronary artery disease; CIED, Cardiac implantable electronic devices; CMD, Coronary microvascular dysfunction; CMR, Cardiac magnetic resonance; CT, Computed tomography; DENSE, Displacement encoding with stimulated echoes; EF, Ejection fractions; ECV, Extracellular volume; FFR, Fractional flow reserve; FT, Feature tracking; GBCA, Gadolinium-based contrast agents; GLS, Global longitudinal strain; HCM, Hypertrophic cardiomyopathy; HF, Heart failure; HFpEF, Heart failure with preserved ejection fraction; HFrEF, Heart failure with reduced ejection fraction; ICD, Implantation of cardioverter-defibrillator; ICM, Ischemic cardiomyopathy; LGE, Late gadolinium enhancement; LV, Left ventricular; MR, Mitral regurgitation; NSF, Nephrogenic systemic fibrosis; RV, Right ventricle; SENC, Strain-encoded imaging; SPECT, Single-photon emission computed tomography; SPINS, Stress CMR Perfusion Imaging in the United States; SCD, Sudden cardiac death.
Introduction
Globally there were 56 million cases of heart failure (HF) in 2019, with an age-standardized prevalence of 712 per 100,000 people [1]. Consequently, HF has resulted in 5.1 million person-years of disability and has disproportionally affected populations with high sociodemographic indexes [1]. Ischemic cardiomyopathy (ICM) and hypertensive heart disease are the most common causes of HF [1]. However, dozens of other etiologies may underlie nonischemic cardiomyopathy, each with unique clinical implications and requiring specific treatments. After the initial diagnosis, patients with HF have a three-fold increased risk of mortality, and 46% survive at 5 years [2]. Unexpectedly, the prognosis is similar between patients with HF with reduced ejection fraction (HFrEF) and HF with preserved ejection fraction (HFpEF) [3]. However, an alarming increase in the incidence of HFpEF has been observed in both men and women [4], and has been strongly associated with rising comorbidity burden [5]. Although echocardiography and invasive hemodynamics are predominantly used to diagnosis HFpEF, according to expert consensus, cardiac magnetic resonance (CMR) is an effective modality for ruling out HFpEF mimics [6]. The complexities of HF diagnosis and management underscore the importance of advanced cardiac imaging in patients with HFpEF.
CMR imaging was developed for clinical use in the 1980s and has since become established as an indispensable and cost-effective tool for the diagnosis of cardiomyopathies. Its versatility enables accurate evaluation of volumes, function, tissue characteristics, perfusion, and strain in a single study with high spatial and temporal resolution. CMR should be considered when echocardiography is inconclusive or windows are inadequate. CMR does not use ionizing radiation, and currently used macrocyclic gadolinium contrast agents are tolerated in renal disease. The number of hospital and outpatient CMRs performed in the US population with Medicare coverage has tripled in the past 10 years [7], thus indicating more routine use. In the past, utilization of CMR was limited by long acquisitions times, a lack of scanner availability, and the need for experienced operators. However, advances in image processing, such as motion correction, free breathing sequences, compressed sensing, and automated segmentation, have increased the throughput of CMR. Consensus guidelines [8, 9] have provided recommendations on the use of CMR in HF (Table 1). The goal of this review is to describe the advantages of CMR in characterizing, monitoring, and determining prognosis in patients with HF (Table 2).
Consensus Guidelines for the Use of CMR in Heart Failure by ESC/HFA and AHA/ACC/HFSA.
Guidelines | CMR recommendations | Class | Level |
---|---|---|---|
2021 ESC/HFA Heart Failure Guidelines | |||
Assessment of myocardial structure and function in patients with poor echocardiogram acoustic windows | 1 | C | |
Characterization of myocardial tissue in patients with suspected infiltrative disease, Fabry disease, inflammatory disease, left ventricular non-compaction, amyloid, sarcoidosis, or iron overload/hemochromatosis | 1 | C | |
Late gadolinium enhancement imaging should be considered in dilated cardiomyopathy to distinguish between ischemic and non-ischemic myocardial damage | 2a | C | |
May be considered for the assessment of myocardial ischemia and viability in patients with coronary artery disease (CAD) who are considered suitable for coronary revascularization | 2b | B | |
2022 AHA/ACC/HFSA Heart Failure Guidelines | |||
Assessment of left ventricular ejection fraction in patients for whom echocardiography is inadequate | 1 | C | |
Can be useful for diagnosis or management of patients with heart failure or cardiomyopathy | 2a | B | |
May be considered for detection of myocardial ischemia, to help guide coronary revascularization in patients with heart failure and coronary artery disease who are candidates for coronary revascularization |
ACC, American College of Cardiology; AHA, American Heart Association; CMR, Cardiac magnetic resonance; ESC, European Society of Cardiology; HFA, Heart Failure Association of ESC; HFSA, Heart Failure Society of America.
Summary of Clinical Uses of CMR Sequences.
CMR sequence | Clinical use |
---|---|
Cine | Chamber size, ejection fraction, myocardial mass, wall thickness |
Phase contrast | Valvular stenosis grade, regurgitant volumes, complex flow patterns |
Perfusion | Ischemia, revascularization targets, microvascular disease |
Late gadolinium enhancement | Cardiomyopathy scar patterns, viability, risk stratification |
Parametric mapping (T1, T2, ECV, and T2*) | Inflammation, myocardial injury, fibrosis, infiltrative diseases |
Strain | Early systolic dysfunction, diastolic dysfunction, atrial myopathy |
CMR, Cardiac magnetic resonance; ECV, extracellular volume.
Size and Function
CMR is considered the gold standard for measurement of systolic function, chamber size, wall thickness, and mass [10]. Compared with two-dimensional (2D) echocardiography, CMR requires fewer geometric assumptions and is not dependent on the quality of acoustic windows to visualize myocardial segments and valves. The ventricular volumes are calculated with Simpson’s summation of discs method, by using a short-axis stack of cine images (Figure 1). Traditional echocardiography estimates ventricular volumes on the basis of only the two-chamber and four-chamber long-axis views by using Simpson’s biplane method. Therefore, function and volumes can differ substantially between two modalities. In addition, echocardiography is based on an assumption that the left ventricular (LV) shape is a prolate ellipsoid in estimation of the mass, but this assumption may not be valid in cardiomyopathy. Because of the accuracy and reproducibility of CMR for measuring function and volume, many experts suggest using this modality for surrogate endpoints in cardiovascular trials [11].

Cine Imaging with Segmentation.
Cine imaging (left) in the short axis for volumetric assessment with automated segmentation of the left ventricular endocardium (red), left ventricular epicardium (green), and right ventricle (teal). The inferior right ventricular insertion point is marked (pink). The volumetric and function measurements are shown (right). CI, Cardiac index; CO, Cardiac output; EDV, End-diastolic volume; EDVI, End-diastolic volume index; EF, Ejection fraction; ESV, End-systolic volume; ESVI, End-systolic volume index; SV, Stroke volume; SVI, Stroke volume index.
Bias associated with echocardiography compared with CMR has been well studied. A meta-analysis of 65 studies comparing echocardiography and computed tomography (CT) versus CMR has indicated that standard 2D echocardiography underestimates left ventricular end-diastolic and end-systolic volume [12]. Using contrast enhancement or three-dimensional acquisition increases the accuracy of echocardiography volumes by decreasing underestimation [12]. These findings suggest that poor endocardial definition and inability to distinguish trabeculations from compact myocardium is a major contributor to bias in 2D echocardiography. The agreement in ejection fractions (EFs) between CMR and echocardiography can be poor in cases with underlying cardiomyopathy. In patients with reduced LV EF on echocardiography, CMR leads to reclassification of function in approximately 25–50% of cases [13–16], thus leading to changes treatment recommendations. In a retrospective study by Champ-Rigot et al. [17], 173 patients with either ICM or dilated cardiomyopathy underwent CMR and 2D echocardiography before implantation of a cardioverter-defibrillator (ICD) for primary prevention. Compared with echocardiography, CMR was a better predictor of death and appropriate device therapy. Regarding overall mortality, only CMR was independently predictive of survival [17]. The right ventricle (RV) can also be difficult to quantify with echocardiography, because of its crescent shape. Consequently, CMR is heavily relied upon to obtain accurate volumetric measurements for the diagnosis of arrhythmogenic right ventricular cardiomyopathy (ARVC). The 2010 Task Force criteria for ARVC use CMR for the measurement of RV end-diastolic volume and EF for major and minor criteria [18]. Comparison studies between CMR and radionucleotide ventriculography in patients with abnormal LV EF have demonstrated good correlation but wide limits of agreement [19, 20].
LV mass measured by CMR is also more accurate and reproducible than echocardiography in both healthy patients and those with cardiomyopathies [21]. LV mass determined by echocardiography can vary as much as 20% in same day studies [22]. Accurate assessment of LV wall thickness and mass is essential for hypertrophic cardiomyopathy (HCM) diagnosis and risk stratification. In a study in 40 sarcomere mutation carriers [23], the CMR maximum wall thickness was approximately 20% higher than that determined with echocardiography, and 10% of patients were reclassified from having normal wall thickness to mild hypertrophy. Therefore, caution is necessary when echocardiography is used to screen for HCM, because this method can miss subtle and early progression of hypertrophy. CMR can also identify morphologic features associated with HCM including myocardial crypts, apically displaced papillary muscles, and elongated mitral leaflets [24]. In patients with suspected HCM, tissue characterization by CMR can also help exclude mimics such as hypertension, amyloidosis, and athletic remodeling [24]. Maximum wall thickness in HCM is a well-known predictor of sudden cardiac death (SCD) [25]. In a study [26] in 190 patients with HCM who underwent CMR and echocardiography within a 6 months of each other, approximately 50% of cases had a ≥10% discrepancy in maximum wall thickness, among which one-third of cases occurred at diagnostic and prognostic cut-offs. Sources of bias in echocardiography include poor windows, inability to detect focal hypertrophy, and inclusion of other structures such as the right ventricle, trabeculations, and papillary muscles [26]. When the European HCM Risk-SCD calculator is used, these discrepancies can lead to an 8% absolute difference in 5-year risk [27]. Regional wall thinning seen on CMR is also a helpful finding in patients with suspected scarring from ischemia [28] or inflammatory cardiomyopathies such as sarcoidosis [29]. CMR is recommended for identifying and measuring the compacted and non-compacted layers in patients with suspected non-compaction cardiomyopathy, because of its high spatial resolution [30].
Phase Contrast and Flow
Phase-contrast imaging with CMR is an excellent tool for the evaluation of valvular HF. Phase-contrast imaging uses magnetic field gradients to encode a velocity map along the through-plane direction, according to the phase of spinning protons. Consequently, the flow and velocity of the protons can be measured at a specific slice plane. Flow imaging is frequently acquired at or above the aortic and pulmonary valve to measure LV and RV stroke volume, respectively, but additional planes can be acquired for complex anatomy and flow patterns.
Although echocardiography continues to be a preferred modality for the initial assessment of valvular disease, CMR should be used in many situations. For stenosis severity, CMR should be considered in patients undergoing surgical evaluation who have contraindications for transesophageal echocardiography, require viability assessment, or have congenital pulmonic stenosis with outflow tract pathology. In general, good agreement exists between CMR and echocardiography in grading stenosis [31, 32]. A major strength of phase contrast CMR in valvular disease is its ability to directly measure regurgitant volumes. Therefore phase contrast CMR is more accurate and reproducible for serial assessments. In chronic aortic regurgitation (AR), progressive LV dilation and remodeling lead to eventual LV dysfunction and poor prognosis. Because patients can remain asymptomatic until late stages of AR, appropriately timing valve replacement before irreversible remodeling occurs can be challenging. In addition to accurate measurements of LV volumetric indices, CMR derived regurgitant volumes and fractions can help reclassify severity and provide incremental prognostic information [33, 34]. A regurgitant fraction greater than 33% has an area under the curve (AUC) of 0.93 for predicting the need for surgery in 3–5 years [34]. Furthermore, CMR can be used to assess the aortic root anatomy to guide valve replacement timing and approach. Mitral and tricuspid regurgitation is usually indirectly quantified by subtracting flow through the semilunar valves from the stroke volume. Similarly to AR, CMR studies of mitral regurgitation (MR) have high accuracy in identifying patients who will develop symptoms or progress to surgery [35]. In cases of severe MR with late systolic, eccentric, or multiple jets, CMR may perform better than echocardiography in identifying adverse outcomes [36]. In addition, CMR can aid in differentiating between primary and secondary MR according to leaflet morphology, scar pattern, and chamber size. Classifying the etiology of MR and anatomy is essential for determining appropriate use of interventions such as transcatheter edge-to-edge repair [37].
Four-dimensional flow (4D flow) is an emerging technique enabling the visualization and quantification of complex flow patterns [38]. With 4D flow, the velocity of blood flow in the LV is encoded in three directions and can be extended to the great vessels. This information is collected and averaged over multiple cardiac cycles. This method is used primarily for research purposes to assess kinetic energy, vortices, eccentricity, and wall shear stress, but it also provides promising insight into how abnormal flow patterns contribute to diastolic dysfunction and adverse outcomes in HF [39].
Perfusion
Stress perfusion CMR plays an important role in newly diagnosed HF and the evaluation for ischemia. Although many modalities are available, CMR offers many advantages, such as high spatial resolution to identify subtle endocardial defects, no ionizing radiation, and lack of artifacts from soft tissue attenuation. Stress CMR is performed by using vasodilators, such as adenosine, regadenoson, or dipyridamole, to induce hyperemia. Gadolinium-based contrast agents (GBCA) are injected during hyperemia, and images are acquired at every heartbeat, as contrast fills the LV cavity and perfuses the myocardium. Areas of normal perfusion have relatively higher concentrations of gadolinium and therefore brighter signal intensity. In addition, the rate of signal change in the myocardium compared with the blood pool is determined by coronary flow and is the core principle underlying quantitative perfusion [40]. Perfusion defects appear as subendocardial areas of hypointensity that match a coronary distribution. Rest images are usually acquired after the vasodilator has worn off or has been reversed. Comparison with rest images is critical for identifying dark rim artifacts, which can mimic perfusion defects. Dark rim artifacts appear as thin subendocardial perfusion defects that are seen in both rest and stress images.
Stress CMR has been shown by numerous studies to have high diagnostic accuracy for ischemia [41]. With invasive angiography as the reference standard, CMR has higher sensitivity (87% vs 67%) than single-photon emission computed tomography (SPECT) but similar specificity (83%) [42]. In addition, the high spatial resolution of stress CMR is unlikely to miss balanced ischemia, which can be a concern with SPECT [43]. Therefore, CMR is an appropriate test to rule out obstructive coronary disease in patients with chest pain, cardiovascular risk factors, and known coronary disease [44]. CMR correlates well with invasive fractional flow reserve (FFR), with a sensitivity of 91% and specificity of 94% for significant stenosis based on FFR <0.75 [45]. Compared with other stress modalities, pooled analyses have demonstrated that the CMR method has among the highest sensitivities and specificities for ischemia on a per-patient basis and per-vessel basis, with invasive FFR as the reference [41]. Quantitative perfusion and the measurement of absolute myocardial blood flow on a pixel-wise basis is increasingly used in clinical practice to complement visual analysis (Figure 2). Quantitative perfusion has been shown to improve the identification of multivessel disease and assess ischemic burden [46–48].
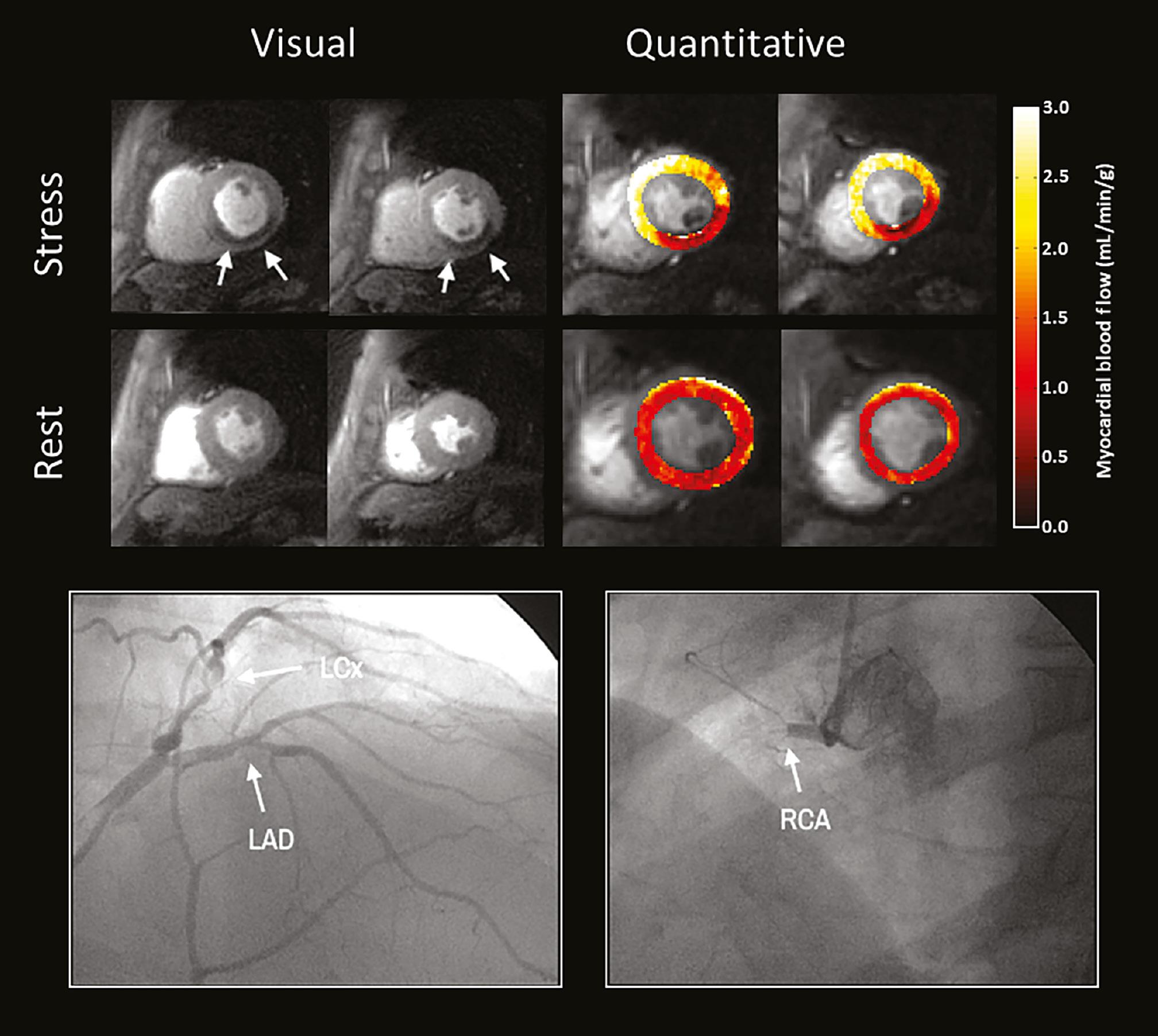
Stress Perfusion Imaging in a Patient with Obstructive Coronary Disease.
A perfusion defect (top) is seen in the short-axis basal to mid inferoseptal, inferior, and inferolateral wall on stress (white arrows), but is not visualized on rest imaging. The quantitative stress flow in this area is reduced to 0.7–1.3 mL/min/g, as compared with the normal myocardium (2.2–2.9 mL/min/g). Coronary angiography (bottom) demonstrated obstructive CAD with 70% stenosis in the left circumflex artery (LCx) and 100% occlusion of the RCA (labeled arrows). The left anterior descending artery (LAD) had a 40% nonobstructive lesion. Adapted from Pan et al.
The high diagnostic performance of stress CMR also translates to better risk stratification and management of patients with HF. In a multicenter study in 9151 patients evaluated for ischemia, abnormal stress CMR has been found to be an independent predictor of mortality, with higher risk in those with LV EF <55% [49]. In a substudy of the Stress CMR Perfusion Imaging in the United States (SPINS) registry including only patients with HFrEF [50], patients with ischemia had an annualized event rate 7.5% for cardiovascular death and myocardial infarction, as compared with 1.8% in patients without ischemia. These findings emphasize the importance of early optimal medical therapy and consideration of revascularization in patients with newly diagnosed HF.
Although obstructive coronary artery disease (CAD) is among the most common causes of HF, coronary microvascular dysfunction (CMD) plays an important role in the development of symptoms [51] and adverse events [52]. CMD can lead to ischemia with non-obstructive coronary artery disease and myocardial infarction with non-obstructive coronary artery disease. In patients with chest pain in the absence of epicardial coronary disease, a myocardial perfusion reserve index below 1.4 is associated with a three-fold increase in major adverse cardiovascular events over 5 years [53]. CMD is also present in patients with HFpEF [54] and nonischemic cardiomyopathies, such as stress cardiomyopathy, dilated cardiomyopathy, HCM, Fabry’s disease, and cardiac amyloidosis [55, 56]. Quantitative stress CMR for detection of CMD has been validated by an invasive coronary flow reserve <2.5, thus achieving an AUC of 0.88 [57]. Early diagnosis of CMD is important, because patients with CMD benefit from aggressive risk factor modifications [58, 59], antianginal agents [60], and targeted therapies for endothelial dysfunction [61, 62].
Late Gadolinium Enhancement
Scarring is prevalent in HF and serves as an important imaging biomarker. Approximately 45% of patients with dilated cardiomyopathy have scars identified through late gadolinium enhancement (LGE) with CMR [63]. The presence of LGE is associated with elevated risk of HF admission, ventricular arrhythmia, and cardiovascular death [63, 64]. In contrast, the absence of LGE is predictive of reverse remodeling and recovery of function [63]. Therefore, LGE is routinely acquired in CMR studies, barring any contraindications. After the administration of GBCA, a delay is necessary before image acquisition to allow for adequate contrast washout from the myocardium. However, in areas of expanded extracellular space from myocyte loss and replacement fibrosis, gadolinium contrast is retained, thereby resulting in enhancement in imaging. The normal myocardium signal is nulled to improve contrast and visualization of diseased myocardium [65].
The pattern of LGE can differentiate between types of cardiomyopathies and exclude potential causes [66]. In ICM, LGE is often seen in the subendocardium, because infarctions begin in that myocardial level (Figure 3), but can extend transmurally to the epicardium in the case of complete and prolonged occlusion of the culprit vessel. A mid-wall stripe of LGE in the interventricular septum in dilated cardiomyopathies is observed in slightly fewer than one-third of patients with this condition [66]. The presence and extent of a mid-wall stripe pattern is an independent predictor of HF admission, SCD, heart transplantation, and death. In cardiac sarcoidosis, the epicardial and mid-myocardial walls of the LV basal septum and lateral segment often develop LGE, which is associated with a 3.5-fold increase in mortality [67]. In chronic Chagas disease, LGE is frequently seen in the apex and inferolateral wall, with focal, transmural, or diffuse involvement [68]. With cardiac amyloidosis, the deposition of proteins such as immunoglobulin light chains and transthyretin into the myocardium results in expansion of the extracellular space and retention of contrast. Consequently, diffuse subendocardial or transmural LGE is frequently observed [69]. In late-stage cardiac amyloidosis, LGE can be so diffuse that images are uninterpretable, because of an inability to correctly null the myocardium. This finding is pathognomonic for cardiac amyloidosis and is a sign of poor prognosis [70]. LGE in the RV insertion points is frequently observed and is considered a nonspecific finding in patients with HCM and pulmonary hypertension [71, 72].

Different Patterns of Late Gadolinium Enhancement Based on Cardiomyopathy.
Examples of short-axis LGE patterns are shown for different cardiomyopathies. Ischemic cardiomyopathy (top left) seen with subendocardial late gadolinium enhancement (LGE) in the basal to mid inferoseptal and inferior myocardium (arrows) consistent with right coronary artery infarction. Dilated cardiomyopathy (top right) seen with a septal mid-wall stripe of LGE (arrows). Hypertrophic cardiomyopathy (middle left) seen with asymmetric hypertrophy and LGE in the septum and inferior right ventricular insertion point (arrows). Myopericarditis (middle right) seen with patchy subepicardial LGE in the anteroseptum and inferolateral walls (arrows). Pericardial effusion and enhancement of the pericardium are also present. Cardiac sarcoidosis (bottom left) seen with extensive subepicardial LGE in the anterolateral, anterior, anteroseptal, inferoseptal, and inferior wall (arrows). Cardiac amyloidosis (bottom right) seen with diffuse transmural LGE. The myocardium could not be nulled correctly.
Scar characterization can guide the management of HF, including revascularization and prevention of ventricular arrhythmias. In ICM, contractile function can be diminished because of myocardial stunning, hibernation, or necrosis [73]. When substantial myocardial necrosis is present, the territory is deemed nonviable, given the irreversible state of remodeling and loss of myocytes. In the case of ischemic LGE patterns, the transmurality should be described in the report to guide the decision of revascularization. Transmural involvement of the myocardium suggests poor viability of the coronary territory and low likelihood of recovery of function after revascularization [74]. In patients with LV EF <35% despite optimal medical therapy, the benefits of ICD placement for primary prevention are clear [9]. However, some patient populations have under-recognized risk of SCD, such as those with sarcoidosis, HCM, and ARVC. CMR with LGE should be considered for identifying scarring and providing additional risk stratification. Scars are hypothesized to be a major substrate for re-entrant and non-re-entrant ventricular arrhythmias, because of altered electrophysiological activity [75]. LGE burden is associated with ventricular arrhythmias, and an approximately 40–50% increase in risk is associated with every 5% increase in LGE to total LV mass [76]. In HCM, an LGE burden greater than 15% of the total LV mass leads to a three-fold increase in SCD and ICD discharge [77]. Therefore, CMR with LGE is recommended in all patients with HCM for evaluation of scar extent. In patients undergoing ventricular ablation, CMR can help electrophysiologists perform preprocedural planning and localization of target lesions. In patients with scar-dependent monomorphic ventricular tachycardia who undergo substrate ablation, LGE-CMR has been shown to decrease procedural, radiation, and ablation times, as well as arrhythmia recurrence [78]. Furthermore, scar burden has incremental value in predicting the response to cardiac resynchronization therapy [79]. Knowing the location of LGE in the LV can also guide placement of the coronary sinus lead [80].
Parametric Mapping
Parametric mapping with CMR enables the quantification of myocardial tissue characteristics. The magnetic relaxation times in the longitudinal (T1) and transverse (T2) directions relative to a static magnetic field generated by the bore of the magnet can be measured on a pixel-wise basis to construct a map of the myocardium. T1 and T2 values can be reported both segmentally and globally [81]. Native T1 times are elevated in cases of myocardial edema, fibrosis, or necrosis, owing to alterations in both the intracellular and extracellular compartments [82, 83]. In contrast, T2 is considered more specific to edema. Increased T2 times have been shown to correlate well with water content in infarcted myocardium of canine models [84]. After the administration of GBCAs, T1 times shorten, primarily on the basis of the volume of distribution of gadolinium in the extracellular space [85]. Therefore, the post-contrast T1 maps should closely resemble the LGE images (Figure 4) [86]. The pre- and post-contrast T1 maps of the myocardium and blood pool can be used to create an extracellular volume (ECV) map after adjustment for hematocrit [87]. This method indirectly measures the interstitial volume fraction of the myocardium by comparing the changes in T1 relaxation of the tissue to that of the plasma. Elevations in ECV can be observed in focal scars, diffuse fibrosis, and infiltrative processes [88]. ECV is reported as a fraction, whereas T1 and T2 times are measured in milliseconds. The normal ranges of T1 and T2 can vary depending on the field strength, scanner vendor, and acquisition sequence [89, 90]. ECV is generally more consistent and reproducible, because it is a normalized metric.

Parametric Mapping in a Porcine Infarction Model.
Multiparametric imaging with cine, T2, pre-contrast T1, post-contrast T1, and late gadolinium (LGE) in the short axis, performed in porcine models before and after left anterior descending artery occlusion. The evolution from acute infarction to chronic remodeling can be seen at day 2, day 30, and day 60 on parametric mapping. Adapted from Lopez et al.
T1 and T2 mapping is an effective modality for detecting inflammation and early necrosis of the myocardium. In patients with suspected myocarditis undergoing CMR and endomyocardial biopsy, T1 and T2 have a diagnostic accuracy of 81% and 80%, respectively, for acute myocarditis [91]. Interestingly, only T2 remains elevated in patients with chronic myocarditis [91]. On the basis of several supporting studies [92], the Lake Louise Criteria for myocarditis were updated in 2018 to include T1-based and T2-based imaging, which increased the sensitivity from 73% to 88% while maintaining a high specificity of 96% [93]. Immune checkpoint inhibitor myocarditis is a rare but potentially fatal complication of immunotherapy and has a mortality rate as high as 40% [94]. In patients with immune checkpoint inhibitor myocarditis, elevated T1 and T2 values are observed in 78% and 43% of patients, respectively [95]. Native T1 is independently associated with adverse cardiovascular outcomes in these patients [95]. Parametric mapping can also be used to assess acute cardiac transplant rejection, with a sensitivity of 93% and specificity of 92% with respect to endomyocardial biopsy [96]. In a single center pilot study in 40 transplant patients [96], those who were randomized to CMR-based surveillance for 52 weeks showed a 94% decrease in endomyocardial biopsies with non-inferior outcomes.
Parametric mapping is also crucial for the quantification of diffuse fibrosis and diagnosis of infiltrative disease. Beyond visible replacement fibrosis on LGE, interstitial fibrosis detected by increased ECV is present in many cardiomyopathies and is associated with poorer HF outcomes. In one study [97], patients with an ECV >30% in segments without LGE have been found to have a 2.5-fold increase in the risk of HF hospitalization and/or death. Elevated global remote ECV remained predictive of adverse outcomes after adjustment for LGE [97]. An ECV above 40% is an infrequent and specific finding seen primarily in cardiac amyloidosis and infarcted myocardium [98]. Because cardiac amyloidosis is caused by the diffuse deposition of proteins in the extracellular space, increased ECV precedes the development of LGE and therefore can enable early diagnosis of the disease [99]. Furthermore, ECV has a higher prognostic odds ratio in cardiac amyloidosis than native T1 and LGE [100]. Fabry disease is a genetic variation that results in the accumulation of glycosphingolipids in various organs, including the heart. Early involvement of myocardium in Fabry disease can be detected with CMR as a decrease in native T1 from lipid overload, with a specificity of 89% in individuals with LV hypertrophy and 99% in individuals without LV hypertrophy [101]. Notably, pseudonormalization of the native T1 occurs as the disease progresses, owing to replacement fibrosis [101]. CMR can also evaluate iron deposition in the myocardium from frequent transfusions or hemochromatosis [102]. Iron deposition creates magnetic inhomogeneities that affect the decay of transverse magnetization, which is measured as T2* relaxation. T2* is inversely correlated with iron load and is predictive of LV dysfunction [103].
Strain
Strain analysis is a growing field in research and clinical practice. Echocardiography studies have demonstrated that strain can identify early myocardial dysfunction and provide incremental information regarding EF [104]. Multiple CMR techniques (Table 3) are available for quantifying strain, including myocardial tagging, feature tracking (FT), tissue mapping, strain-encoded imaging (SENC), and displacement encoding with stimulated echoes (DENSE) [105]. Most of these modalities are used primarily for research purposes and have not been clinically applied. Nevertheless, strain analysis with CMR is an attractive option in the near future for assessing HF.
Overview of Cardiac Magnetic Resonance Strain Methods.
Strain method | Overview of method |
---|---|
Myocardial tagging | Applies an in-plane tagging gradient to produce dark lines that form a grid on the myocardium. The deformation of the grid can be visualized to assess deformation. |
Strain-encoded imaging (SENC) | Applies a tagging gradient in the through-plane direction to a series of myocardial planes, to measure how they compress together throughout the cardiac cycle. |
Displacement encoding with stimulated echoes (DENSE) | Uses radiofrequency pulses and gradients to encode displacement into the signal phase of the image, to provide pixel-wise vectors of magnitude and displacement. |
Phase velocity mapping | Uses gradients to encode tissue velocity maps based on the phase of the signal, and integrates the velocities in three directions to calculate myocardial displacement. |
Feature tracking | Post-processing technique that detects features of user-defined myocardial segments and tracks the displacement between successive images in the same plane. |
FT strain analysis is a promising newer method that assesses strain on cine images according to user-defined contours and can be completed offline with dedicated software. Global longitudinal strain (GLS) and global circumferential strain measured by FT CMR closely correlate with speckle-tracking echocardiography findings [106]. FT strain can be assessed on atria and ventricles (Figure 5). However, FT strain can be assessed only within the acquired imaging plane and cannot fully capture the myocardial deformation in three dimensions. FT strain may be useful for assessing diastolic dysfunction. In patients with HFpEF who underwent invasive pressure-volume loop measurements, LV GLS by FT CMR has been found to be highly correlated with Tau (r = 0.817, P < 0.001), a measure of LV relaxation and a marker of diastolic dysfunction [107]. In addition, an LV GLS ≥−8% according to FT CMR has been shown to predict HF hospitalization and cardiovascular mortality in individuals with HFpEF [108]. Abnormal left atrial strain with FT CMR is also associated with impaired LV filling, diminished exercise capacity, and risk of HF [109, 110]. In a study involving 120 patients with suspected HFpEF, left atrial FT strain and size have been shown to have a high diagnostic accuracy significantly better than that of LV and RV FT strain parameters (P < 0.01) [111].

Feature Tracking Strain Analysis of the Left Atrium and Ventricle.
The left atrium (orange), left ventricular endocardium (red), and left ventricular epicardium (green) are segmented in the two-chamber and four-chamber views. Strain is color-coded in the left ventricle and tracked in the left atrium (maroon wavy lines). Longitudinal strain plots are shown for the left atrium (upper right) and left ventricle (lower right).
Limitations
Several limitations of CMR should be addressed. First, substantial costs are associated with CMR, including upgrading scanners, training technologists, and hiring experienced readers. In addition, indirect expenses are associated with the opportunity costs of non-cardiac MRI studies that could have been performed, the time required for patients to travel to CMR capable centers, and potential downstream testing. However, several studies [112–117] have shown that CMR is a cost-effective modality, particularly for decreasing unnecessary downstream testing and interventions in patients with CAD. In patients with HFrEF, CMR has a major impact on clinical decision-making in approximately 65% cases, with 30% receiving an entirely new diagnosis and 52% resulting in a change in management [118]. A paucity of clinical trials have demonstrated the incremental financial value of CMR compared with echocardiography, because of the need for different cost and value assumptions across health systems. Nevertheless, several studies [119, 120] have used economic and cost-effectiveness models to draw conclusions. Schneider et al. [119] have compared the cost effects of echocardiography and CMR strain assessment with SENC in patients with HF, for both the payer and hospital. Their model has indicated that CMR with SENC contributes to 37% lifetime savings ($24,647 vs $39,097) for payers with better contribution margins for the hospital than echocardiography. Many experts would agree that greatest clinical benefits of CMR come from scar detection in all cardiomyopathies, which is not possible with other modalities including echocardiography. In a systematic review [120] by the National Institute of Health Research, cost-effectiveness models have been used to compare CMR versus other testing pathways for ischemic cardiomyopathy. In two separate modeling scenarios, contrast CMR and stress CMR were the dominant strategy over SPECT and echocardiography, respectively.
Second, additional attention is needed for patients with irregular heart rates and cardiac implantable electronic devices (CIED). Patients with pacemakers and ICDs should be screened before their study, because of the risk of the magnetic field causing temporary reprograming of the CIED, artifactual electrocardiogram changes detected by the device, and heating of lead tips that can result in myocardial injury [121]. Because many cine images are usually acquired during a breath hold over multiple heart beats, irregular heart rates from arrythmias can contribute to improper gating and reconstruction artifacts. Real-time sequences allow for acquisition of cine images independently of electrocardiogram gating and with free breathing, thus bypassing the problems associated with irregular rhythms, but at the cost of lower spatial or temporal resolution. Nevertheless, the diagnostic quality is usually better with real-time imaging in patients with atrial fibrillation [122]. CIED also contributes to substantial metal artifacts in CMR that degrade the diagnostic value of the study. In fact, parametric mapping is not reliable in patients with CIED and therefore should not be reported [123]. Left-sided ICD generators are most problematic because of their proximity to the heart. Several strategies can be applied to decrease metal artifacts from CIED, including maximizing the distance between the generator and the heart by raising the ipsilateral arm, adjusting pulse sequence parameters, or using “wideband” techniques [123].
Finally, in certain clinical scenarios, alternative modalities should be considered over CMR. Patients with suspected transthyretin cardiac amyloidosis or cardiac sarcoidosis should undergo radionucleotide imaging with disease-specific tracers [124]. In addition, cardiac CT should be considered in patients requiring detailed evaluation of the coronary, valve, and atrial appendage [125]. Studies have shown excellent correlation between CT and CMR measurements of LV volumes and ejection fractions [12]. However, radionucleotide imaging and CT introduce radiation risks to patients. Claustrophobia is another barrier to CMR, for which many experts suggest mild sedation and prone positioning [126].
GBCAs should also be avoided in specific cases, because of the risk of nephrogenic systemic fibrosis (NSF) and tissue retention [127]. Because heavy metals can be toxic, gadolinium contrast is chelated to improve its safety profile during intravenous injection. Therefore, GBCAs can be classified according to molecular configuration (linear vs macrocyclic) and charge (ionic vs non-ionic). NSF is a rare complication of GBCAs that occurs in patients with advanced kidney failure and results in progressive multiorgan fibrosis. The risk of NSF depends on the type of agent used and is most frequently associated with older linear agents that are no longer in use in most countries. In contrast, cases of NSF with newer macrocyclic agents are extremely rare, and therefore these agents are considered safe [127]. According to consensus statements [128] from the American College of Radiology and National Kidney Foundation, screening patients with severe kidney disease and withholding GBCAs with very low risk of NSF is not recommended, given the harm associated with delaying clinical indicated MRIs. Gadolinium has also been shown to deposit in other tissues including the brain [127]. The retention of the gadolinium in the brain is associated with GBCA type, but no evidence suggests harmful effects. However, the use of GBCAs should be limited in pregnant patients, because of potential associations with adverse fetal outcomes [129].
Future Directions
In recent years, the intersection between CMR and artificial intelligence has markedly grown and heralded a new wave of innovations in HF management. Automated pipelines are being developed to select images and analyze them in a fast and accurate manner without manual intervention [130, 131]. CMR is also an ideal modality for radiomics, because of the vast number of sequences that can be used. Radiomics is a type of quantitative analysis based on features within images, such as shapes, textures, and signal profiles. In a study from the UK Biobank [132], radiomics has been applied to patients who underwent CMR and experienced adverse cardiovascular events, such as atrial fibrillation, HF, myocardial infarction, and stroke. The addition of radiomic features to vascular risk factors significantly improved the prediction of atrial fibrillation (AUC 0.67 vs 0.76, P < 0.05) and HF events (AUC 0.73 vs 0.83, P < 0.05) in patients. Because numerous latent features in CMR images can be used to differentiate among cardiomyopathy subtypes, many experts have proposed using CMR to phenomap patients with HF, particularly HFpEF [133, 134]. In patients with ischemic cardiomyopathy, comprehensive CMR analysis combined with unsupervised learning can identify clusters according to infarct size and the degree of remodeling [135]. These clusters are associated with treatment response to revascularization [135]. CMR parameters can also be combined with cardiac biomarkers to identify mechanisms associated with HFpEF [136].
Conclusion
CMR is a powerful imaging modality that should be considered in patients with HF. In a single CMR examination, accurate volumetric and functional assessment, flow and perfusion imaging, tissue characterization, and strain analysis can be obtained for a patient. A wealth of evidence supports the use of CMR for diagnosing cardiomyopathies, performing risk stratification of patients, and providing guidance in HF management. With increasing technological availability and improvements, providers should consider incorporating CMR into clinical practice as a standalone test, a complementary modality, or a means for monitoring cardiac diseases over time.