Background
Diabetic cardiomyopathy (DCM), a major complication of diabetes, substantially contributes to the global public health burden [1, 2]. This multifaceted condition is a distinct clinical entity characterized by myocardial dysfunction and abnormal cardiac metabolism in people with diabetes, independently of coronary artery disease and hypertension [3]. The pathogenesis of DCM is a multifactorial process in which oxidative stress and apoptosis play crucial roles.
Oxidative stress, characterized by an imbalance between the accumulation of reactive oxygen species (ROS) and the body’s antioxidative defenses, is exacerbated under diabetic conditions [4]. The elevated glucose in diabetes leads to excessive production of ROS. These reactive molecules consequently inflict considerable damage on crucial cellular components, including lipids, proteins, and DNA. This damage culminates in cell death through a process known as apoptosis, thus providing a critical pathophysiological link in the progression of DCM [5, 6].
Investigations of the molecular mechanisms underlying DCM have focused on two key proteins: methionine sulfoxide reductase A (MsrA) and calcium/calmodulin-dependent protein kinase II (CaMKII). MsrA, an antioxidant enzyme, repairs proteins damaged by oxidative stress, thus preserving cellular function under adverse conditions [5, 6]. In contrast, CaMKII, particularly when oxidatively modified (ox-CaMKII), has been implicated in DCM pathogenesis by promoting myocardial apoptosis and fibrosis, thereby impairing cardiac function [7, 8]. Emerging evidence highlights a role of MsrA in mitigating ox-CaMKII activation in cardiovascular diseases, thereby suggesting a protective effect. However, the relevance of this pathway to DCM remains to be explored further [9].
Berberine (BBR), an isoquinoline alkaloid extracted from Rhizoma coptidis, has received considerable attention for its potential cardioprotective properties. Accumulating evidence highlights its beneficial effects in alleviating oxidative stress, inflammation, and apoptosis in cardiovascular diseases [10, 11]. However, the specific role and underlying mechanisms of BBR in the context of DCM remain elusive.
Therefore, the current study was aimed at exploring the effects and mechanistic action of BBR in DCM, specifically focusing on its influence on MsrA expression and cardiac CaMKII oxidation.
Methods
Mouse Model of Diabetic Cardiomyopathy
The DCM model in mice was established as previously described [12]. The experimental animals were purchased from Beijing Weitong Lihua Experimental Animal Technology Co., Ltd., and the animal experiment was approved by the Ethics Committee of Tianjin First Central Hospital. Male C57 mice 8 weeks of age were divided into control, DCM, and BBR groups. Mice in the control group were maintained on a normal chow diet throughout the study. Mice in the DCM and BBR groups were subjected to a high-fat diet (45% fat, 0.25% cholesterol) for 6 weeks, then tested for glucose intolerance and insulin resistance. At 14 weeks, both the DCM and BBR groups exhibited elevated blood glucose levels after glucose and insulin injection, thus indicating impaired glucose tolerance and insulin sensitivity. Diabetes was induced with streptozotocin (75 mg/kg, Sigma, USA) injection. Mice with fasting blood glucose levels ≥11.1 mM were included in the DCM and BBR groups. Both the DCM and BBR groups continued to receive the high-fat diet for an additional 15 weeks, for a total of 22 weeks. After establishment of the DCM model, mice in the DCM group were administered physiological saline, whereas those in the BBR group were administered BBR (20 mg/kg × day) via gavage for 8 weeks. All mice were monitored until 30 weeks.
Echocardiographic Measurement
Mice were anesthetized with isoflurane, after which transthoracic echocardiography was conducted with a Vevo2100 imaging system (VisualSonics, Toronto, Canada). This procedure was performed by echocardiographers who were blinded to the group assignments of the mice. Measurements of left ventricular end-diastolic diameter (LVEDD), left ventricular ejection fraction (LVEF), fractional shortening (FS), left ventricular posterior wall thickness (LVPW), and interventricular septum thickness (IVS) were obtained through M-mode echocardiography in the left ventricular long-axis view. Pulsed Doppler imaging in four-chamber view was used to measure the early (E) and late (A) diastolic mitral flow velocities, from which the E/A ratio was calculated. Tissue Doppler imaging in the four-chamber view was used to measure the early (E´) and late (A´) diastolic mitral annular velocities, and the E´/A´ ratio was subsequently determined.
Histology
Heart weight (HW) and tibial length (TL) were measured in all mice and used to calculate the HW/TL ratio. Hearts were freshly excised and immediately fixed in 10% formalin. After fixation, the tissues were embedded in paraffin and sectioned into 4 μm thick slices. Hematoxylin and eosin (HE) staining was performed to visualize cardiomyocyte morphology. Masson’s trichrome staining was used to detect myocardial fibrosis, and the fibrotic area was calculated as a percentage of the total myocardial area. Wheat germ agglutinin staining was conducted to delineate the cell boundaries of cardiomyocytes, thus enabling calculation of the cross-sectional area of the cardiomyocytes.
TUNEL Staining
Myocardial sections (4 μm thick) underwent terminal deoxynucleotidyl transferase-mediated dUTP nick end-labeling (TUNEL) staining. After deparaffinization and antigen retrieval with citrate buffer, sections were treated with 3% H2O2 for 10 min and blocked with 5% BSA for 30 min at 37 °C. Apoptosis was detected with an In Situ Cell Death Detection Kit and TMR red (Roche, Germany). After being washed with PBS, sections were stained with 4′,6-diamidino-2-phenylindole (DAPI) for 10 min at 37 °C. Images were captured with a fluorescence microscope (Ni-E, Nikon, Japan). The apoptosis ratio, expressed as the percentage of apoptotic cells to total myocardial cells, was calculated.
Flow Cytometry Analysis of Cardiomyocyte Apoptosis
Freshly excised mouse hearts were subjected to enzymatic digestion to isolate cardiomyocytes. The isolated cells were then stained with an apoptosis detection kit specifically designed for flow cytometry. The staining procedure involved a combination of fluorescently labeled annexin V and a propidium iodide solution. After staining, the cells were analyzed with a flow cytometer set up to detect the fluorescence of annexin V and propidium iodide. The flow cytometry data were analyzed with appropriate software to determine the percentage of apoptotic cells, calculated as the number of annexin V positive cells (early and late apoptotic cells) divided by the total number of cells.
Detection of Superoxide Dismutase and Malondialdehyde in Mouse Blood
Fresh blood samples were collected from the mice and immediately processed for the detection of superoxide dismutase (SOD) and malondialdehyde (MDA). For SOD detection, blood samples were subjected to a specific SOD assay measuring the dismutation of superoxide radicals, performed according to the manufacturer’s instructions. For MDA detection, a Bioxytech LPO-586 assay kit was used. This assay is based on the reaction of MDA, a biomarker of lipid peroxidation, with N-methyl-2-phenylindole, thus forming a stable chromophoric product. The absorbance of this product was measured at 586 nm with a spectrophotometer. The MDA concentration was calculated with a standard curve according to the manufacturer’s instructions.
Western Blot Analysis
Protein extracts from mouse hearts and cells were subjected to SDS-PAGE separation and subsequently transferred onto polyvinylidene fluoride membranes. The membranes were then incubated with various primary antibodies at 4 °C overnight and subsequently incubated with suitable secondary antibodies for 1 hour at room temperature. The relative protein expression levels were determined by normalization to GAPDH expression. This experiment used primary antibodies to BCL2-associated X protein (BAX) (Proteintech, 50599-2-Ig, 1:2000), B-cell lymphoma 2 (Bcl2) (Proteintech, 68103-1-Ig, 1:2000), MsrA (Proteintech, 14547-1-AP, 1:500), CAMKII (Proteintech, 12666-2-AP, 1:1000), and ox-CAMKII (Gene Tex, AB_2887447, 1:1000).
Statistical Analysis
The statistical results for all figures and tables are presented as mean ± SD. GraphPad Prism software (version 7.0, CA) was used for one-way or two-way ANOVA with post hoc Tukey’s test or unpaired test (two-tailed), when appropriate, or to calculate the Pearson correlation coefficient and the P-value (two tailed). Differences were considered significant at P < 0.05.
Results
Body Weight, Blood Glucose, and Serum Lipid Profiles
Body weight (BW), fasting blood glucose (FBG), total cholesterol (TC), and triglycerides (TG) were significantly higher in the DCM group than the control group. However, no significant differences in BW, FBG, and serum lipid levels were observed between the BBR group and DCM group (Table 1). These findings suggested that the introduction of BBR did not significantly alter these metabolic parameters in the context of DCM.
Body Weight, Blood Glucose, and Serum Lipid Profiles in Mice.
Control (n = 6) | DCM (n = 6) | BBR (n = 6) | |
---|---|---|---|
BW (g) | 26.65 ± 1.26 | 35.61 ± 2.31* | 34.65 ± 1.85 |
FBG (mmol/L) | 7.51 ± 1.21 | 29.41 ± 2.13* | 28.24 ± 5.21 |
TC (mmol/L) | 3.15 ± 0.97 | 4.12 ± 1.04* | 4.31 ± 0.81 |
TG (mmol/L) | 1.55 ± 0.28 | 1.99 ± 0.35* | 2.01 ± 0.42 |
HDL-C (mmol/L) | 0.82 ± 0.62 | 0.80 ± 0.32 | 0.99 ± 0.25 |
LDL-C (mmol/L) | 2.18 ± 0.23 | 2.34 ± 0.56 | 2.21 ± 0.47 |
Data are shown as mean ± SD; *P < 0.05 vs. control group. BW, body weight; FBG, fasting blood glucose; TC, total cholesterol; TG, triglycerides; HDL-C, high-density lipoprotein cholesterol; LDL-C, low-density lipoprotein cholesterol.
Improved Cardiac Remodeling and Function in Berberine-Treated Diabetic Mice
Echocardiographic evaluations indicated notable differences in cardiac remodeling and function among groups. In the DCM group, the LVEDD, LVPW, and IVS were significantly greater than those in the control group. In contrast, the LVEF, FS, E/A ratio, and E´/A´ ratio were significantly lower in the DCM group than the control group (Figure 1B–I).
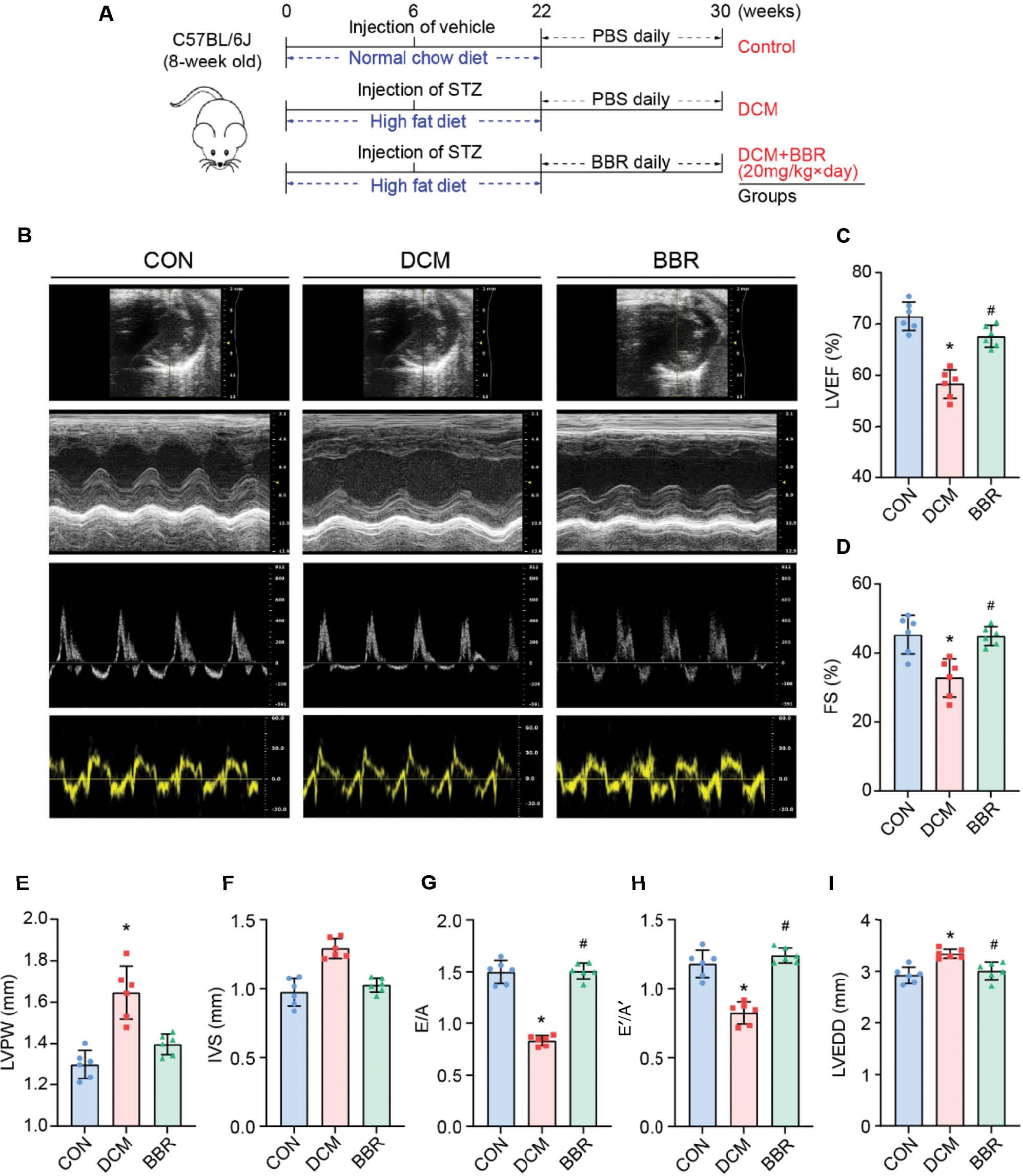
Experiment timeline and echocardiographic measurements in three groups of mice.
(A) Experiment timeline in three groups of mice. (B) Representative echocardiographic images in mice: (1) Two-dimensional echocardiogram showing left ventricular short-axis view; (2) M-mode echocardiogram showing left ventricular dimensions; (3) Pulse-wave Doppler echocardiogram depicting diastolic mitral flow; (4) Tissue Doppler echocardiogram displaying mitral annular velocities. (C) Measurements of left ventricular ejection fraction (LVEF) in mice. (D) Measurements of left ventricular fractional shortening (FS) in mice. (E) Measurements of left ventricular posterior wall (LVPW) thickness in mice. (F) Measurements of interventricular septum (IVS) thickness in mice. (G) Measurements of the ratio of early to late diastolic mitral flow velocities (E/A) in mice. (H) Measurements of the ratio of early to late diastolic mitral annular velocities (E´/A´) in mice. (I) Measurements of left ventricular end-diastolic diameter (LVEDD) in mice. *P < 0.05 vs. the control group; #P < 0.05 vs. the DCM group; n = 6.
However, these alterations in cardiac remodeling and function were significantly ameliorated in the BBR group. Specifically, the BBR group exhibited a reversal of the echocardiographic changes observed in the DCM group, with decreased LVEDD, LVPW, and IVS measurements, and increased LVEF, FS, E/A, and E´/A´ ratios (Figure 1B–I). These findings suggested that BBR treatment effectively improves cardiac remodeling and both systolic and diastolic function in diabetic mice.
Alleviation of Cardiac Hypertrophy and Fibrosis in Berberine-Treated Diabetic Mice
Histological examination of myocardial cells was conducted with HE staining. In the control group, the cardiomyocytes demonstrated a well-ordered arrangement, with uniformly distributed myocardial fibers. In contrast, the DCM group exhibited some thickened cardiomyocytes with irregular and deeply stained nuclei, thus indicating compensatory enlargement. In contrast, the BBR group displayed only slightly enlarged cardiomyocytes, and nuclei appearing relatively normal (Figure 2A).
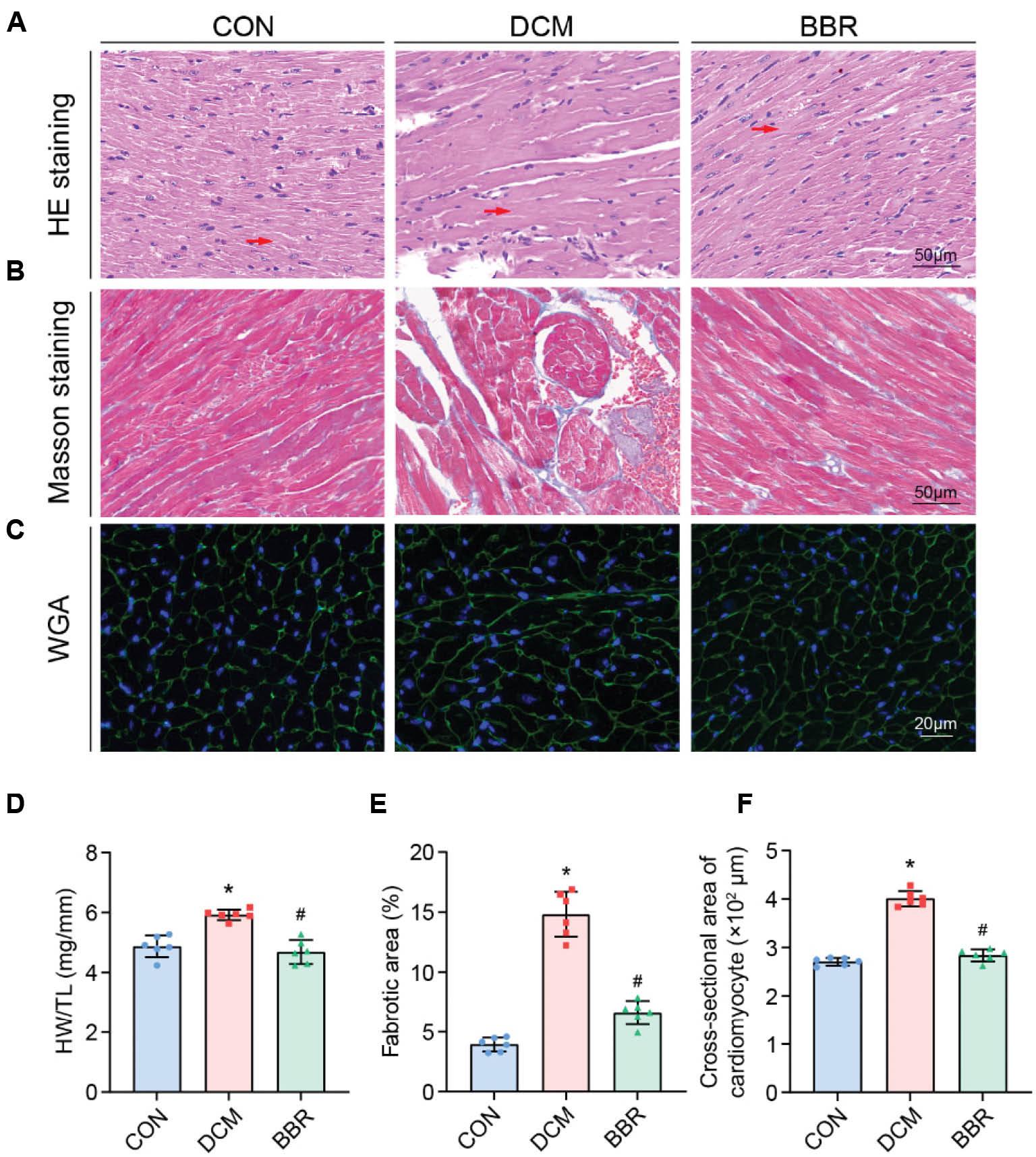
Histological staining in three groups of mice.
(A) Representative HE staining of myocardial cross-sections; (B) Representative Masson’s trichrome staining of cardiac short-axis cross sectional areas. The arrows indicate the myocardial cells. (C) Representative WGA staining of cardiac short-axis cross sectional areas; (D) Quantitative analysis of heart weight/tibial length (HW/TL) ratio in mice. (E) Quantification of the fibrosis area in mice. (F) Quantification of the cross-sectional area of cardiomyocyte in mice. *P < 0.05 vs. the control group; #P < 0.05 vs. the DCM group; n = 6.
Measurements of HW and TL were collected from all mice, and the HW/TL ratio was calculated. In the DCM group, the HW/TL ratio was significantly greater than that in the control group, thus indicating cardiac hypertrophy (Figure 2D). This hypertrophy was accompanied by increases in myocardial fibrosis, as evidenced by Masson’s trichrome staining, and in the cross-sectional area of cardiomyocytes, as determined by wheat germ agglutinin staining (Figure 2E–F). However, these pathological changes were significantly ameliorated in the BBR group. Specifically, the BBR group exhibited a decrease in the HW/TL ratio, indicating diminished cardiac hypertrophy (Figure 2D). Furthermore, the extent of myocardial fibrosis and the cross-sectional area of cardiomyocytes were lower in the BBR group than the DCM group (Figure 2E, F). These findings suggested that BBR treatment effectively mitigates cardiac hypertrophy, myocardial fibrosis, and cardiomyocyte enlargement in mice with DCM.
Berberine Alleviates Cardiomyocyte Apoptosis and Restores the BCL2/BAX Ratio
We evaluated the effect of BBR on cardiomyocyte apoptosis in DCM mice with TUNEL staining and flow cytometry. We observed a significantly greater apoptosis ratio, indicating elevated cardiomyocyte apoptosis, in the DCM group than the control group (Figure 3A, B). However, the apoptosis ratio was significantly lower in the BBR group than the DCM group, thereby suggesting that BBR mitigated cardiomyocyte apoptosis in DCM mice (Figure 3A, B). Flow cytometry analysis further corroborated these findings, revealing a higher percentage of apoptotic cells in the DCM group than the control group (Figure 3C, D). However, the BBR group exhibited a lower percentage of apoptotic cells than the DCM group (Figure 3C, D) [13].
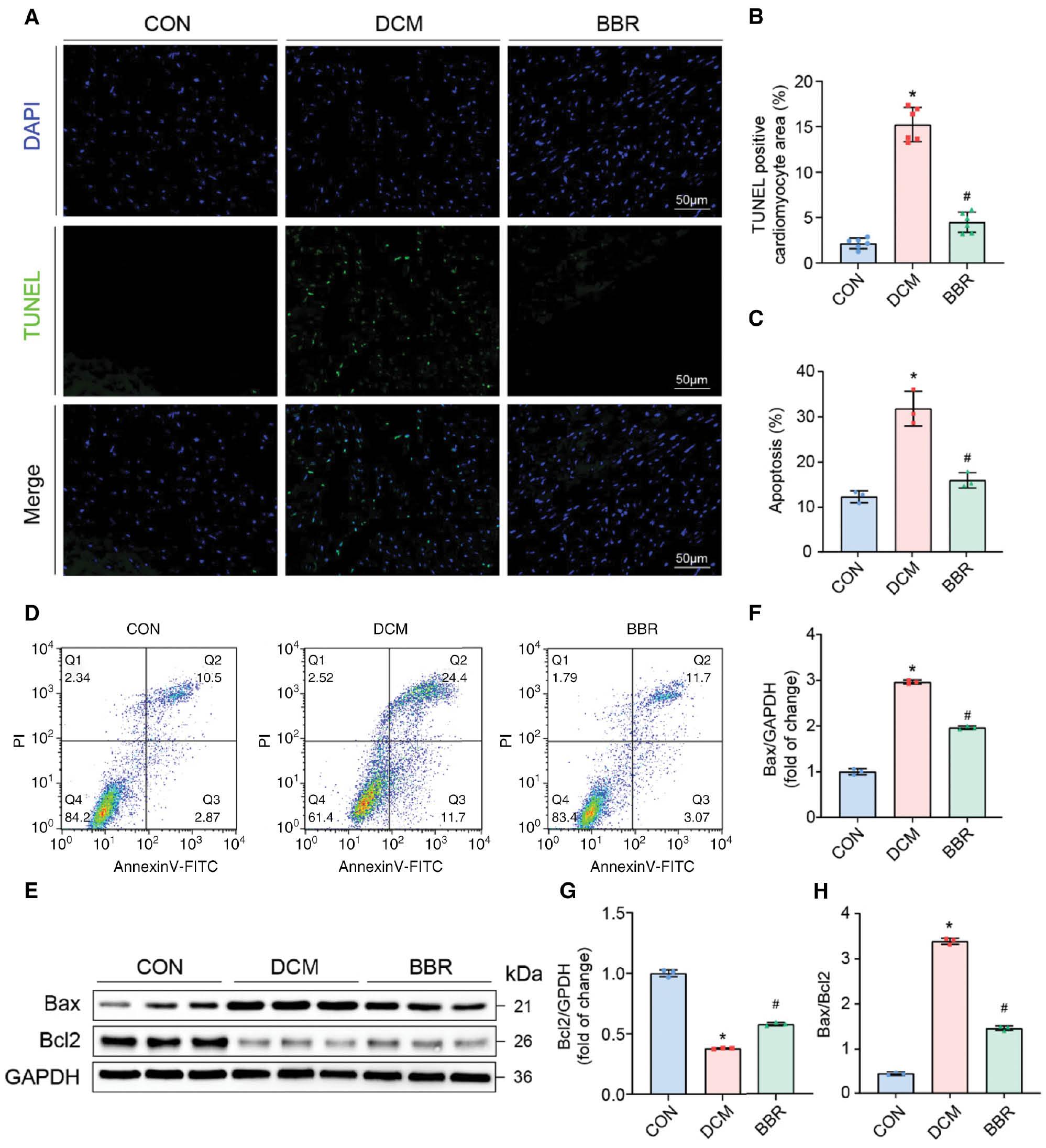
BBR reduces DCM-induced apoptosis.
(A) Representative TUNEL-positive cardiomyocyte staining in mice. (B) Quantification of the TUNEL positive cardiomyocyte area in mice, n = 6. (C) Quantitative flow cytometry analysis of apoptotic cardiomyocytes, n = 3. (D) Representative scatter plots from flow cytometry analysis of isolated mouse cardiomyocytes. (E-G) Protein expression of BAX and BCL2 in heart tissues were determined by Western blot with quantitative analysis of band density, n = 3. *P < 0.05 vs. the control group; #P < 0.05 vs. the DCM group.
Moreover, we investigated the effects of BBR on the protein expression of BCL2-associated X protein (BAX), a proapoptotic factor, and B cell lymphoma 2 (BCL2), an anti-apoptotic factor. In the DCM group [14], we observed elevated BAX protein expression and diminished BCL2 expression, thus leading to an elevated BAX/BCL2 ratio indicative of the activation of the mitochondrial cell death pathway (Figure 3E–G). Remarkably, BBR substantially reversed these changes, thereby demonstrating the potential of this treatment to modulate the expression of key proteins involved in apoptosis (Figure 3E–G).
Berberine Alleviates Oxidative Stress in Diabetic Cardiomyopathy
We further explored the effects of BBR on oxidative stress in DCM mice. In the DCM group, we observed diminished expression of the antioxidant enzyme SOD and elevated expression of MDA, a marker of lipid peroxidation (Figure 4A, B). These findings indicated elevated oxidative stress in DCM mice. However, BBR treatment significantly increased SOD expression and decreased MDA expression, thus suggesting that BBR alleviated oxidative stress in DCM mice (Figure 4A, B). DHE staining indicated that BBR substantially inhibited DCM-induced ROS levels (Figure 4G–H).
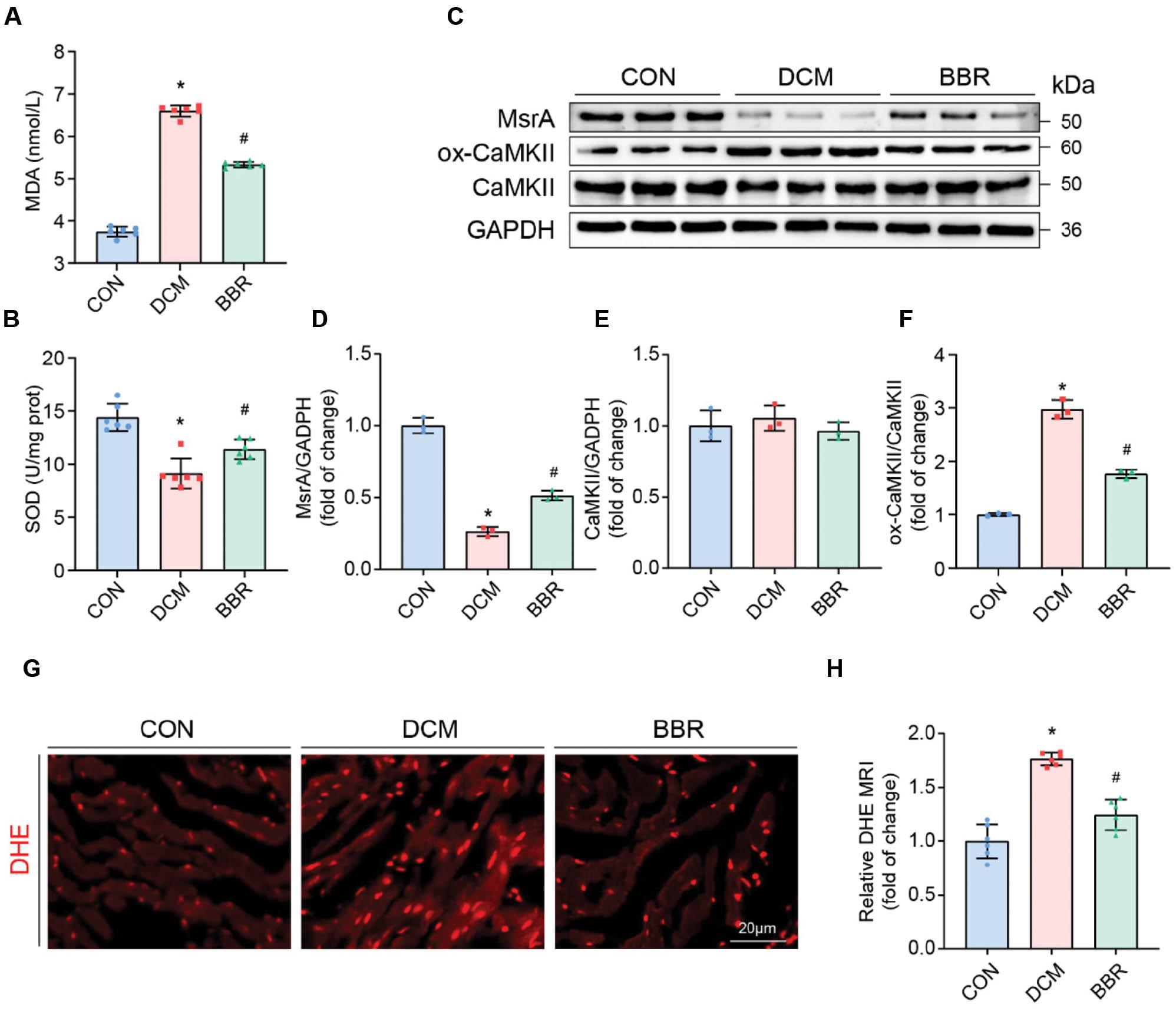
BBR concurrently inhibits ox-CaMKII levels and enhances MsrA expression, reducing DCM-induced oxidative stress.
(A) Serum malondialdehyde (MDA) levels, n = 6. (B) Serum superoxide dismutase (SOD) levels, n = 6. (C-E) Protein expression of MsrA, ox-CaMKII and total-CaMKII in heart tissues were determined by Western blot with quantitative analysis of band density, n = 3. (F, G) Representative DHE staining and quantitative analysis of DHE MFI of myocardial cross-sections, n = 6. *P < 0.05 vs. the control group; #P < 0.05 vs. the DCM group.
Importantly, BBR treatment increased the expression of MsrA, an enzyme that reduces oxidized CaMKII, thereby potentially mitigating the harmful effects of oxidative stress. This finding was in line with those from recent studies highlighting the role of MsrA in modulating the oxidative state of CaMKII, a key player in oxidative stress and cardiac dysfunction [15]. Furthermore, following BBR treatment, we observed a decrease in the expression of ox-CaMKII, a protein associated with oxidative stress and cardiac dysfunction [16] (Figure 4C–F). These changes suggested a complex interplay of stress responses in the context of BBR treatment.
Discussion
DCM, a consequence of dysregulated glucose and lipid metabolism in diabetes mellitus, remains a major challenge, because of the complexity of its pathogenesis and the difficulty in developing targeted interventions [1, 17]. BBR, a naturally occurring isoquinoline alkaloid, has been demonstrated to have substantial cardioprotective potential, by markedly decreasing myocardial infarct size and mitigating ventricular arrhythmia, according to animal studies [18]. In this study, we extended these findings by showing that BBR treatment significantly improved cardiac function and decreased cardiac remodeling in mice with DCM, thus revealing its potential as an effective therapeutic approach for DCM. Although previous research had established the role of BBR in DCM [19], we performed deeper investigation of the mechanistic action of BBR. We explored how BBR modulates the MsrA and CaMKII pathways, thereby influencing cardiomyocyte apoptosis and oxidative stress in the context of DCM. This in-depth mechanistic investigation enhances understanding of BBR’s therapeutic potential and provides valuable insights that may guide the development of novel therapeutic strategies for DCM.
Oxidative stress, characterized by an imbalance between the production of ROS and the body’s antioxidant defenses, is a key player in the pathogenesis of DCM [20]. In diabetes, elevated glucose levels lead to excess production of ROS, thus resulting in oxidative stress, which in turn substantially damages essential cellular components. This oxidative stress instigates cell death through a process known as apoptosis. Moreover, in diabetes, ROS further exacerbate apoptosis through several pathways [21], including the activation of complex metabolic routes, such as the DAG-PKC-NADPH oxidase pathway, direct oxidative damage to cellular components, triggering of inflammatory responses, and dysregulation of cellular processes such as autophagy [22, 23]. Our findings indicated that BBR effectively decreases oxidative stress and apoptosis in cardiomyocytes affected by DCM, thus reinforcing earlier research that established the beneficial effects of BBR on cardiac function [24, 25].
Our study demonstrated that BBR increases the expression of MsrA in the myocardium, and decreases the oxidation of CaMKII, myocardial oxidative stress, and apoptosis in a mouse model of DCM. These findings contribute to the growing body of evidence indicating the role of CaMKII, a serine/threonine kinase, in various cardiovascular diseases, particularly in the context of diabetes [26–28]. Previous studies have underscored the importance of CaMKII in endothelium-derived relaxation and eNOS activity in type 2 diabetes, and its role in hyperglycemia-induced apoptosis of retinal capillary endothelial cells [29, 30]. Moreover, the absence of CaMKII after cardiac injury has been associated with diminished cardiac remodeling and with reversal of symptoms in heart failure; therefore, CaMKII inhibition may be a potential treatment [31–33]. Cardiac-specific CaMKIIδ gene deletion has been associated with a decrease in ischemia–reperfusion induced infarct formation and myocardial cell death via the NFκB-signaling pathway [34, 35]. However, the effects of CaMKII activity on oxidative stress and apoptosis in DCM have not been extensively explored.
Our findings suggest a novel mechanism of action of BBR in the context of DCM. MsrA, a key component of the antioxidant defense system, counteracts oxidative stress by reducing methionine sulfoxide back to methionine [36]. Dysregulation of MsrA has been implicated in the pathogenesis of cardiovascular disorders, which are associated with elevated oxidative stress [37]. Previous studies have indicated that MsrA, a ubiquitous protein oxidation repair enzyme, decreases CaMKII oxidation, thereby inhibiting transformation of CaMKII into a perpetually active state, decreasing its overactivation, and mitigating its contribution to cellular damage [9, 38]. In a mouse model deficient in MsrA, cardiomyocytes have been found to exhibit elevated sensitivity to oxidative stress, thus leading to cardiac dysfunction and perturbations in calcium dynamics [39]. In this study, BBR appeared to exert its cardioprotective effects by increasing MsrA expression, and consequently decreasing CaMKII oxidation and inhibiting CaMKII oxidation-associated myocardial cell apoptosis. The role of BBR in modulating MsrA expression and CaMKII oxidation presents a promising avenue for further investigation. Future studies should be aimed at elucidating the precise molecular pathways involved, and assessing the potential therapeutic implications of these findings in the treatment of DCM.
Conclusion
In conclusion, our study demonstrated the cardioprotective effects of BBR in a mouse model of DCM, through increasing MsrA expression, and decreasing CaMKII oxidation, myocardial oxidative stress, and apoptosis. These findings suggest a novel mechanism of BBR’s action, thereby potentially offering a new therapeutic approach for DCM.