Significance Statement
This study describes the expression patterns and functions of cardiac PEDF during cardiac development, thus laying a foundation for further studies on the effects of cardiac PEDF in acquired heart disease.
Introduction
Cardiovascular diseases (CVDs), such as hypertension, coronary atherosclerosis, heart failure (HF) and arrhythmia, are characterized by high incidence and mortality rates, and they are the main factors leading to death in the global population [1, 2]. Several important genetic mutations have been associated with the development of congenital heart disease, and many abnormally expressed proteins are closely associated with the development of various acquired heart diseases [3–7]. Notably, multiple genes associated with acquired heart disease simultaneously influence cardiac development, and the abnormal expression of their encoded proteins may have potential impact on cardiac structure and function during cardiac development. Therefore, clarifying the spatiotemporal expression characteristics of proteins involved in cardiac development, and their effects on cardiac structure and function during cardiac development, would help more clearly define their roles in acquired heart disease.
Pigment epithelium-derived factor (PEDF) is a secreted protein with a molecular weight of 50 kD that consists of 418 amino acids; its gene is located at 17p13.1, and contains 8 exons and 7 introns [8–11]. Although PEDF is a member of the serine protease superfamily, it does not possess protease inhibition function [8, 12]. PEDF is widely distributed in a variety of tissues, and is particularly highly expressed in the liver and adipose tissue; however, its expression in cardiac tissues has been less clear [13, 14]. In addition, PEDF has been shown to be a multifunctional protein whose major physiological functions include inhibition of angiogenesis, neuroprotection, metabolic regulation, and antioxidation, as well as clear protective effects in CVDs such as myocardial infarction, ischemia-reperfusion and atherosclerosis [8, 15–22]. However, whether PEDF is involved in the cardiac developmental process remains unclear.
In the present study, on the basis of analysis of the correlations between cardiac PEDF and multiple heart diseases, we determined that cardiac and aorta PEDF expression is higher than that in the liver. We additionally confirmed that cardiac PEDF expression significantly decreases after birth, and this decrease is accompanied by altered cardiomyocyte sublocation characteristics. Furthermore, by using histological staining and echocardiography, we confirmed that PEDF deficiency does not significantly affect cardiac structure, cardiac function and vascular hemodynamics during cardiac development.
Methods
GEO Dataset Analysis
Data from the GSE760, GSE19210, GSE30428, GSE4105, GSE47495 and GSE775 data sets were used to analyze the correlation between cardiac PEDF expression and the occurrence and development of various heart diseases [23–27]. All samples in the above datasets are from animal models, and only the sample data from the disease model group and the corresponding control group were subjected to further analysis. Specific sample information can be found in Supplementary Table 1.
Animals
C57BL/6J wild-type mice were purchased from the Laboratory Animal Center of Sun Yat-sen University. PEDF knockout (C57BL/6J background) mice were kindly provided by Prof. Guoquan Gao (Zhongshan School of Medicine, Sun Yat-sen University) and Prof. Xia Yang (Zhongshan School of Medicine, Sun Yat-sen University).
Male and female mice 30 postnatal days of age were used to analyze PEDF expression differences among tissues. Fetal, suckling or adult mice at different developmental time points were used to analyze altered cardiac PEDF expression at different developmental times. Eight-week-old PEDF knockout mice and wild-type littermate controls were used to assess the effects of PEDF deficiency during cardiac development on cardiac structure, cardiac function and vascular hemodynamics.
All animals were housed under a 12-hour light-dark cycle at 18–21 °C, and given free access to water and food. All animals were fasted for 12–16 hours before sampling and testing.
Western Blotting
The tissues (three samples per group) were lysed with RIPA buffer (Beyotime, Shanghai, China) for total protein extraction. The protein concentration was determined with a BCA protein assay kit (Millipore, Bedford, MA, USA) according to the manufacturer’s protocol. Equal amounts of protein were subjected to SDS-PAGE for electrophoresis, transferred to 0.45-μm PVDF membranes (Millipore, Bedford, MA, USA) and immunoblotted with antibodies to PEDF (MAB1059, Millipore, Bedford, MA, USA). The bands were quantified in the ImageJ software program (National Institutes of Health, Bethesda, MD, USA).
Immunohistochemistry
Heart samples were fixed in 4% paraformaldehyde overnight, then subjected to conventional dehydration and slicing. Subsequently, heart sections (5 μm) were blocked with 3% hydrogen peroxide and then incubated at 95 °C for 10 min in citrate buffer (P0083, Beyotime, Shanghai, China); blocking steps were performed with QuickBlock™ Blocking Buffer (P0260, Beyotime, Shanghai, China) according to the manufacturer’s instructions. After incubation with primary antibodies at 4 °C overnight, the sections were incubated with secondary antibodies (G1210, Servicebio, Wuhan, Hubei, China) at 37 °C for 30 min. Visualization was accomplished with 3,3 N-diaminobenzidine tertrahydrochloride (G1211, Servicebio, Wuhan, Hubei, China). Sections were counterstained with hematoxylin (G1004, Servicebio, Wuhan, Hubei, China). Primary antibodies to PEDF (ab227295, Abcam, Cambridge, MA, USA) were used. The mean density was quantified from three or four individual mice, and four to six fields per mouse in the Image-Pro Plus software program (Media Cybernetics, Bethesda, MD, USA).
Histological Staining
Histological staining was performed on frozen sections. The heart tissues were fixed in 4% paraformaldehyde (pH 7.4) for 24 h, dehydrated with a sucrose gradient, embedded in Tissue-Tek OCT compound (Sakura Finetek, Tokyo, Japan) and serially sectioned at 5 μm. Subsequently, standard hematoxylin and eosin (HE) staining and Masson’s trichrome staining were performed as described in previous studies [28]. Images were captured with a Leica microscope (DFC700T, Leica, Germany).
Echocardiography and Vascular Ultrasound
Echocardiography and vascular ultrasound were performed on mice with a Vevo 2100 imaging system (Visual Sonics, Toronto, Canada), as described in previous studies [28]. In detail, the mice were placed in a closed box filled with 1.5% isoflurane. After being anesthetized, the mice were immediately placed on a 37 °C thermostat to maintain normal body temperature and were maintained on 0.5% isoflurane to prevent them from awakening before the end of the imaging process. For image acquisition, a 30-MHz probe was used. Ultrasound images of cardiac short-axis and the aorta diameter (Ao Diam) were captured in M-Mode. Ultrasound images of the mitral valve blood flow, peak velocity in aortic valve (AV Peak Vel) and peak velocity in the descending aorta (Desc Ao Vel) were captured in PW Doppler-mode. The heart rate in all mice was controlled to 450–550 bpm during imaging.
Statistical Analysis
All analyses were performed in Statistical Package for the Social Sciences version 19.0 (SPSS, Chicago. IL, USA). The data are expressed as mean ± SD. Statistical differences between two groups were analyzed with unpaired Student’s t tests, and differences between multiple groups of data were analyzed with one-way ANOVA. In all statistical comparisons, a P value<0.05 was used to indicate a statistically significant difference.
Results
Cardiac PEDF Expression is Associated with the Occurrence and Development of Various Acquired Heart Diseases
To clarify the correlation between cardiac PEDF expression and heart diseases, we screened several heart gene expression datasets of rodent models of acquired heart disease from the GEO database, and analyzed the correlation between cardiac PEDF expression and disease progression. Analysis of the GSE760 data set indicated that the expression of cardiac PEDF was significantly greater in the mouse model of dilated cardiomyopathy (DCM) than in control mice (Figure 1A). Analysis of the GSE19210 dataset indicated that the expression of cardiac PEDF in hypertensive rats with HF was significantly higher than that in hypertensive rats without HF (Figure 1B). Analysis of the GSE30428 dataset demonstrated that the expression of cardiac PEDF was significantly higher in the mouse model of right ventricular (RV) hypertrophy than in sham operated mice (Figure 1C). Analysis of the GSE4105 data set revealed that the expression of cardiac PEDF was significantly higher at 2 and 7 days after ischemia-reperfusion (I/R) than in sham operated rats (Figure 1D). Analysis of the GSE47495 dataset indicated that the expression of cardiac PEDF was significantly greater in rats with large area myocardial infarction (MI) than sham operated rats, but did not significantly differ in mice with small versus moderate areas of MI (Figure 1E). Analysis of the GSE775 data set showed that, compared with that in sham operated mice, the expression of cardiac PEDF did not change significantly within 48 hours after MI, increased significantly 1 week after MI, and returned to normal 8 weeks after MI (Figure 1F).
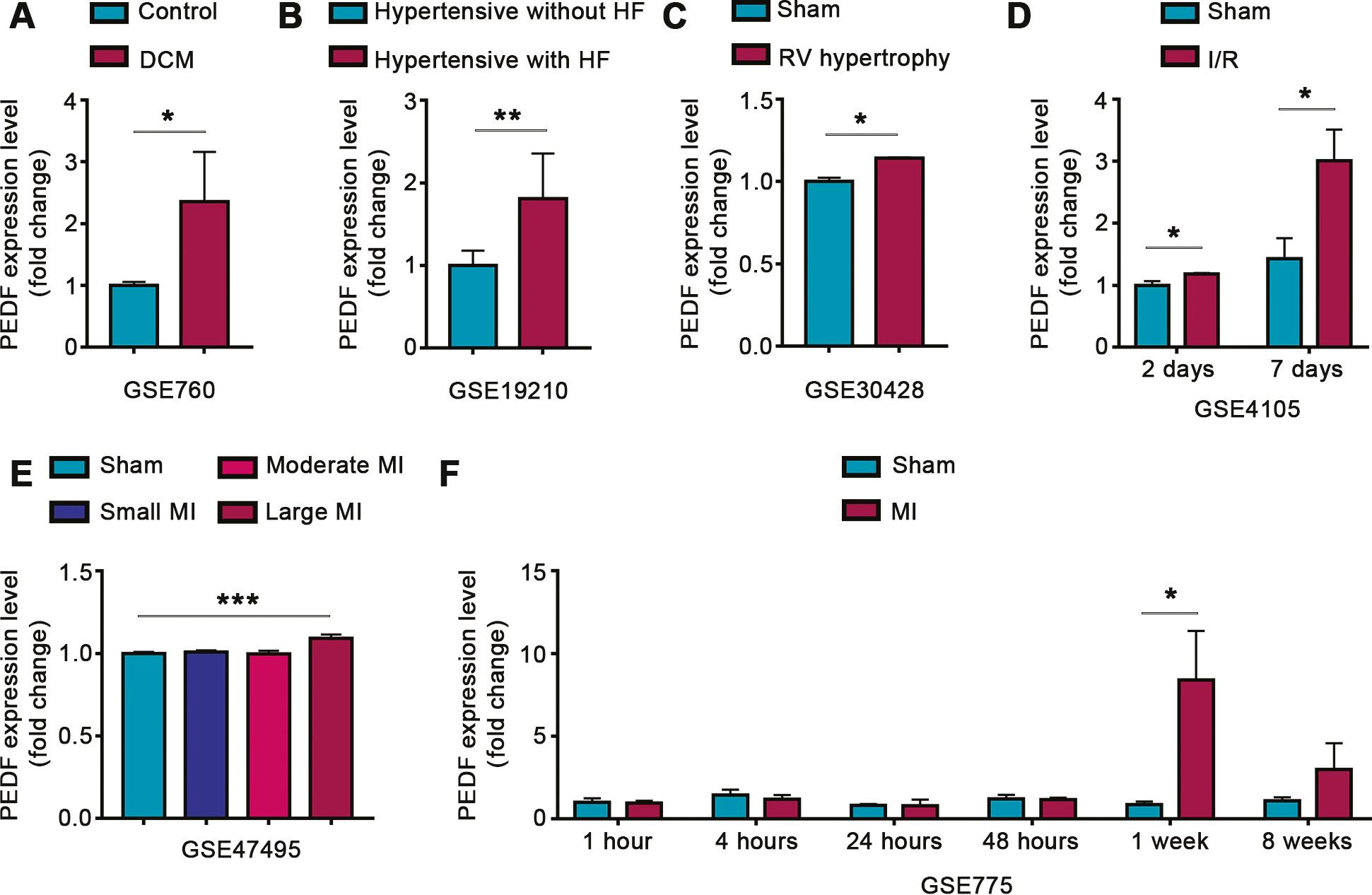
Correlation between Cardiac PEDF Expression and Heart Disease.
A. Cardiac PEDF expression between DCM and control mice, on the basis of the GSE760 data set. B. Cardiac PEDF expression between hypertensive rats with HF and hypertensive rats without HF, on the basis of the GSE19210 data set. C. Cardiac PEDF expression between RV hypertrophy and sham mice, on the basis of the GSE30428 data set. D. Cardiac PEDF expression between I/R and sham rats, on the basis of the GSE4105 data set. E. Cardiac PEDF expression in MI rats with different infarct sizes, on the basis of the GSE47495 data set. F. Cardiac PEDF expression in MI mice at different times after infarction, on the basis of the GSE775 data set. DCM, dilated cardiomyopathy; HF, heart failure; RV, right ventricular; I/R, ischemia-reperfusion; MI, myocardial infarction. Data are expressed as mean ± SD. *, P<0.05; **, P<0.01; ***, P<0.001.
These results confirmed that the expression of cardiac PEDF is associated with the progression of various acquired heart diseases.
PEDF is Highly Expressed in the Heart and Aorta in Postnatal Mice
To clarify the distribution of PEDF expression in each tissue, we selected male and female mice 30 days after birth and examined PEDF expression in several tissues including the liver, mesentery, heart, lungs, kidneys, skeletal muscle, spleen and aorta. We assessed the differences in PEDF expression in these tissues compared with the liver. In male mice, PEDF expression in the mesentery, lungs and spleen was significantly lower than that in the liver. PEDF expression in the kidneys and skeletal muscle did not significantly differ from hepatic PEDF expression, whereas PEDF expression in the heart and aorta was significantly higher than that in the liver (Figure 2A, B). In addition, similar expression profiles were found in female mice: lung, skeletal muscle and spleen PEDF expression was significantly lower than hepatic PEDF expression; mesenteric and kidney PEDF expression did not significantly differ from hepatic PEDF expression; and heart and aorta PEDF expression was significantly higher than that in the liver (Figure 2C, D).
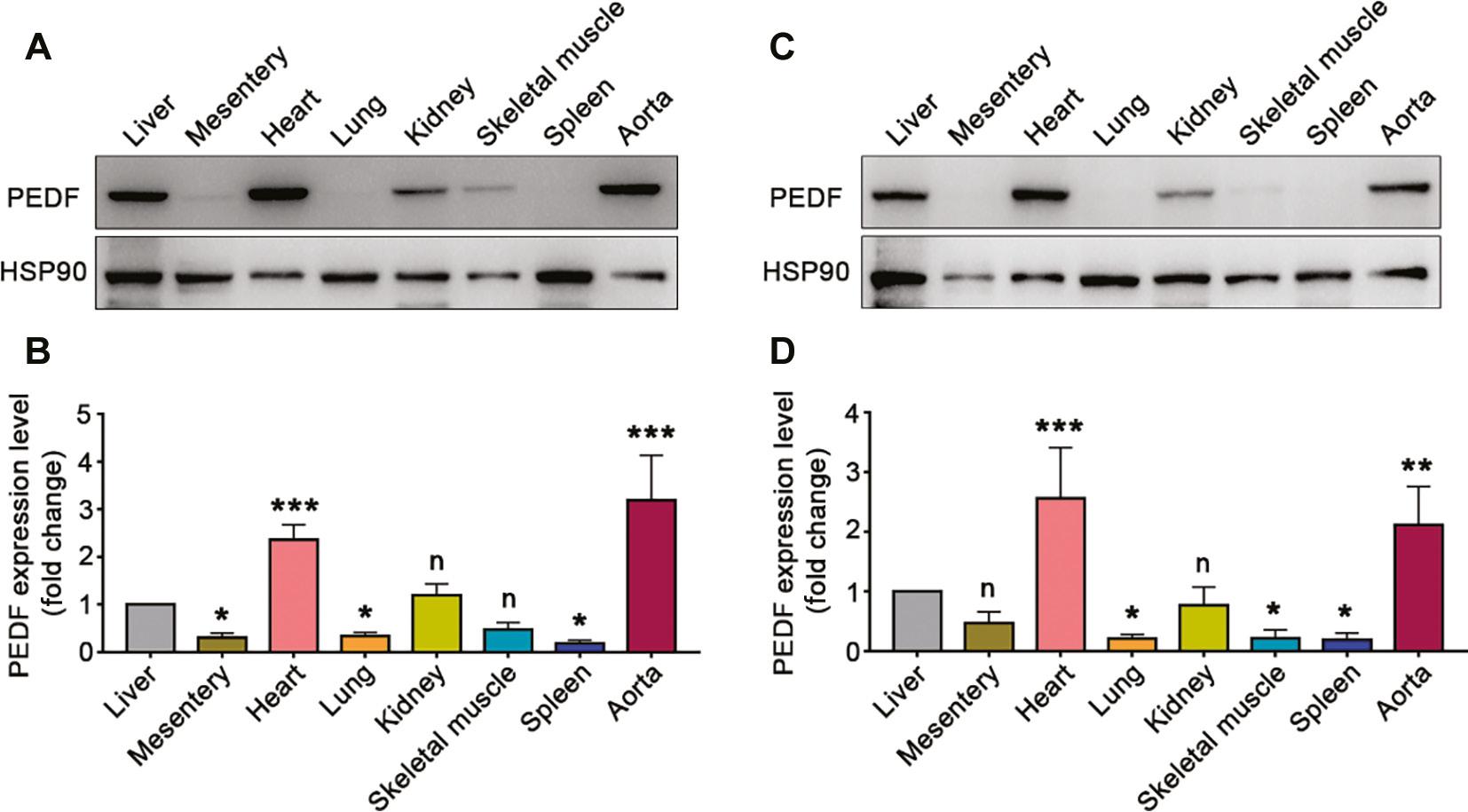
Expression of PEDF in Different Tissues.
A–B. PEDF expression in different tissues of male mice at postnatal 30 days (n=3). C–D. PEDF expression in different tissues of female mice at postnatal 30 days (n=3). Data are expressed as mean ± SD. *, P<0.05; **, P<0.01; ***, P<0.001.
These results confirmed that PEDF is highly expressed in the heart and aorta in postnatal mice, and excluded the influence of sex.
Cardiac PEDF Expression Decreases Significantly After Birth and Shows Changes in Subcellular Localization
To clarify the dynamic changes in cardiac PEDF expression during cardiac development, we selected cardiac tissues at different developmental times and performed immunohistochemical staining to observe the changes in PEDF expression and subcellular localization characteristics. The expression of PEDF in mouse hearts at the embryonic stage and postnatal 30 days was similar, and was widely distributed in the cytoplasm (Figure 3A, B). At postnatal 30 days and postnatal 60 days, the expression of PEDF had significantly decreased in mouse hearts, notably in the cytoplasm (Figure 3A, B).
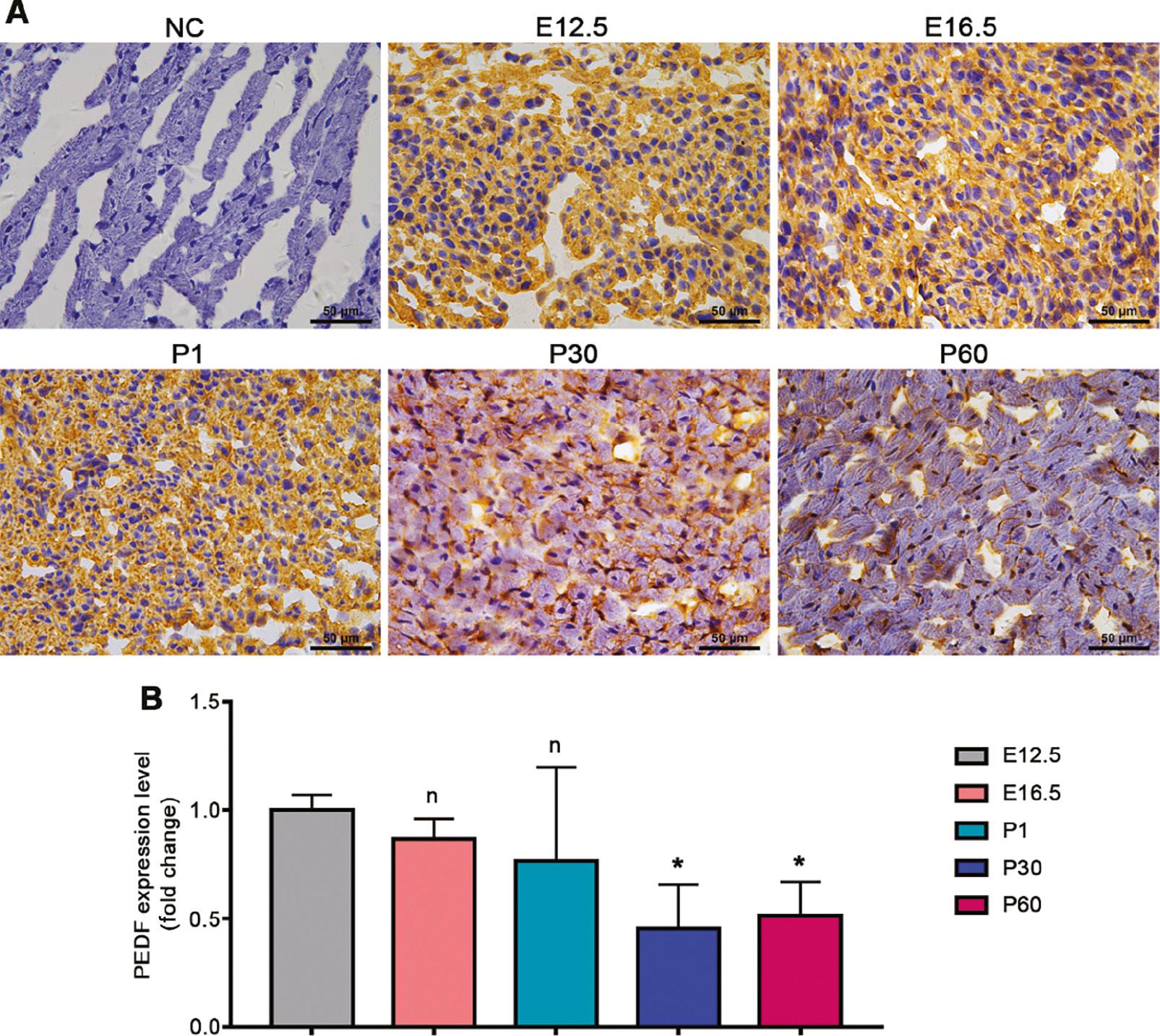
Cardiac PEDF Expression at Different Time Points in Cardiac Development.
A. Immunohistochemical staining results. B. Quantitative results of immunohistochemical staining (n=3–4). Data are expressed as mean ± SD. *, P<0.05.
These results confirmed that cardiac PEDF expression significantly decreases after birth and is characterized predominantly by a decrease in cytoplasmic PEDF abundance.
PEDF Deficiency During Cardiac Development has No Significant Effects on Cardiac Structure
To further clarify the effects of PEDF deficiency on cardiac structure during cardiac development, we evaluated cardiac structure changes in PEDF global knockout mice and wild-type littermate controls. The heart weight and heart weight/tibia length ratio of PEDF knockout mice did not significantly differ from those in the wild-type littermate control mice (Figure 4A, B). HE staining indicated no significant differences in heart structure between PEDF knockout mice and wild-type littermate control mice (Figure 4C). Masson’s trichrome staining also indicated that the hearts in PEDF knockout mice and wild-type littermate control mice showed no significant differences in fibrosis (Figure 4D).
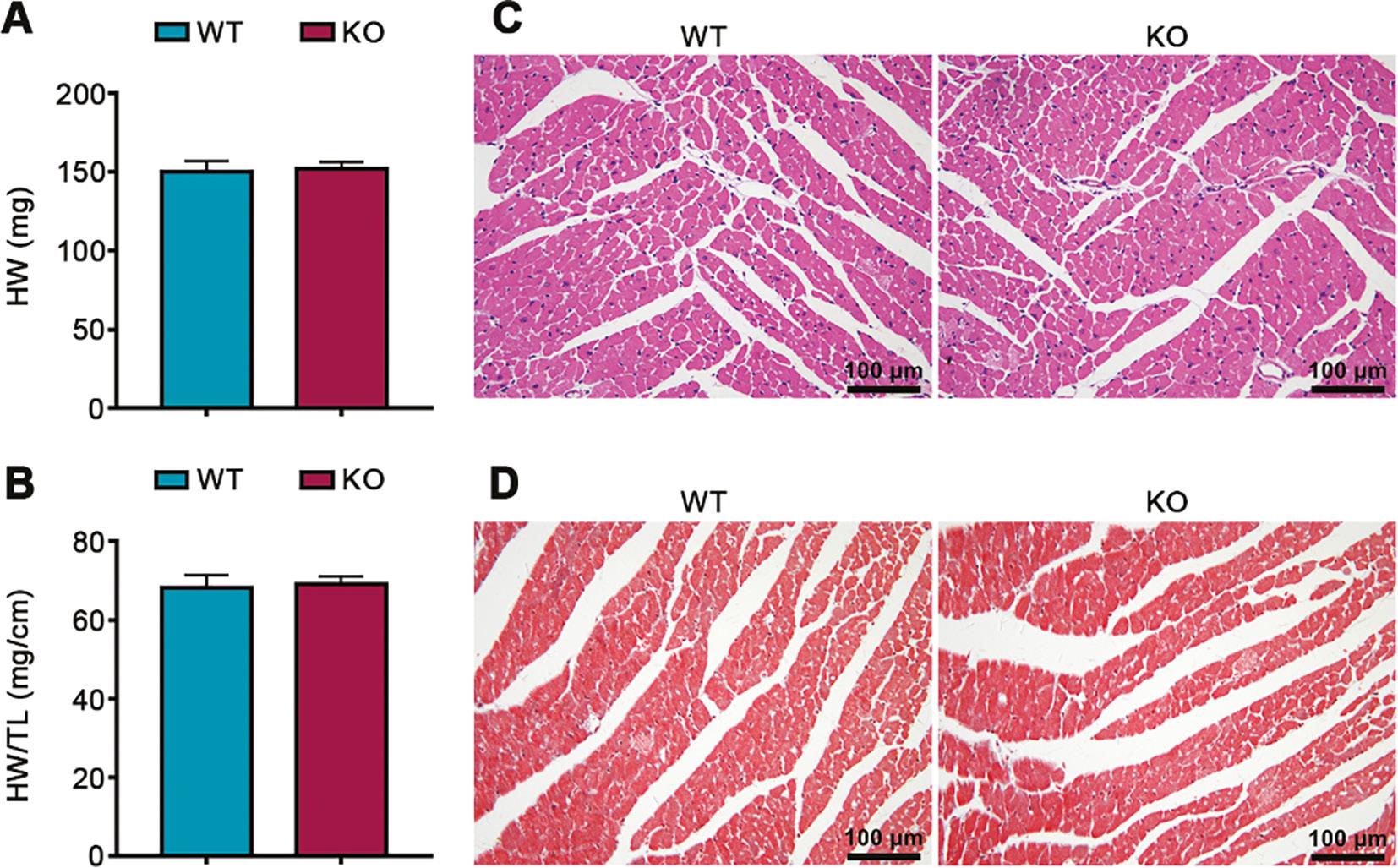
Effects of PEDF Deficiency on Cardiac Structure During Cardiac Development.
A. Heart weight (n=5). B. Heart weight/tibia length ratio (n=5). C. HE staining. D. Masson’s trichrome staining.
These results confirmed that PEDF deficiency during cardiac development does not significantly affect cardiac structure.
PEDF Deficiency During Cardiac Development Does Not Significantly Affect Cardiac Function
By echocardiography, we evaluated the effects of PEDF deficiency on both cardiac diastolic function and cardiac systolic function during cardiac development. Cardiac diastolic function did not significantly differ between PEDF knockout mice and wild-type littermate controls, as reflected by an unchanged E/A peak ratio, isovolumetric contraction time (IVCT) and isovolumic relaxation time (IVRT) (Figure 5A–D). In addition, the ejection fraction (EF), fractional shortening (FS), left ventricular internal diameter (LVID) at the end of systole, left ventricular anterior wall (LVAW) and left ventricular posterior wall (LVPW) at the end of diastole and end of systole did not significantly differ between PEDF knockout mice and wild-type littermate controls, and only the LVID at the end of diastole was somewhat diminished (Figure 5E–K). These results suggested that PEDF deficiency during cardiac development has a very weak effect on cardiac systolic function.
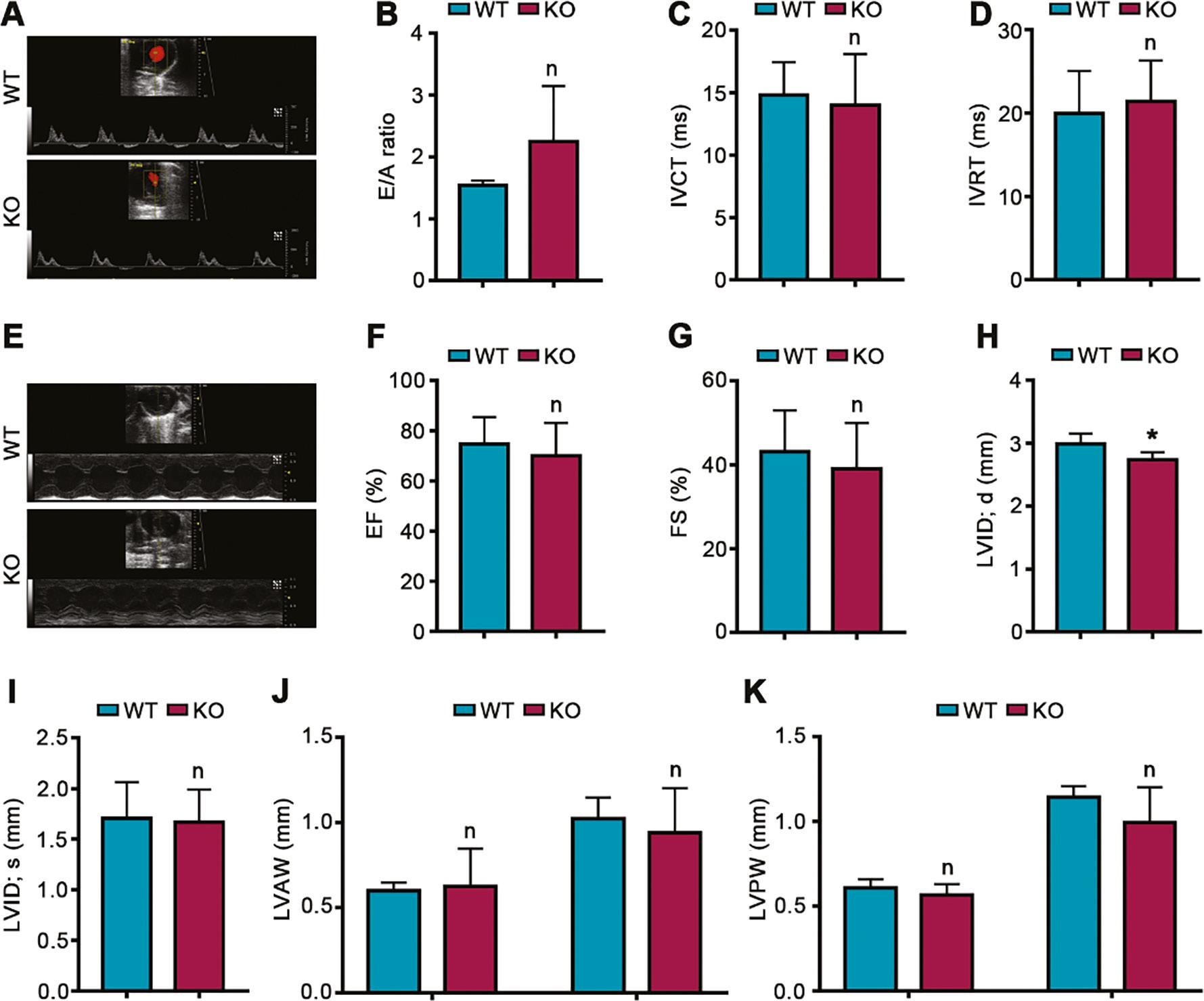
Effects of PEDF Deficiency on Cardiac Function During Cardiac Development.
A. Mitral valve rheography. B. E/A ratio (n=4). C. IVCT (n=4). D. IVRT (n=4). E. Echocardiography images in short-axis. F. EF (n=4). G. FS (n=4). H. LVID at the end of diastole (n=4). I. LVID at the end of systole (n=4). J. LVAW (n=4). K. LVPW (n=4). IVCT, isovolumetric contraction time; IVRT, isovolumic relaxation time; EF, ejection fraction; FS, fractional shortening; LVID, left ventricular internal diameter; LVAW, left ventricular anterior wall; LVPW, left ventricular posterior wall. Data are expressed as mean ± SD. *, P<0.05.
These results additionally confirmed that PEDF deficiency during cardiac development does not significantly affect cardiac function.
PEDF Deficiency During Cardiac Development Does Not Significantly Affect Vascular Hemodynamics
Given that our results confirmed that PEDF is highly expressed in the aorta and that normal aortic development is closely associated with the development of heart disease, we further evaluated the effects of PEDF deficiency during cardiac development on aortic hemodynamics. Vascular ultrasound results indicated no significant differences in the end-diastolic Ao Diam and the end-systolic Ao diam between PEDF knockout mice and wild-type littermate controls (Figure 6A–C). Our results also demonstrated that the AV Peak Vel and the Desc Ao Peak Vel did not significantly differ between PEDF knockout mice and wild-type littermates (Figure 6D–G).
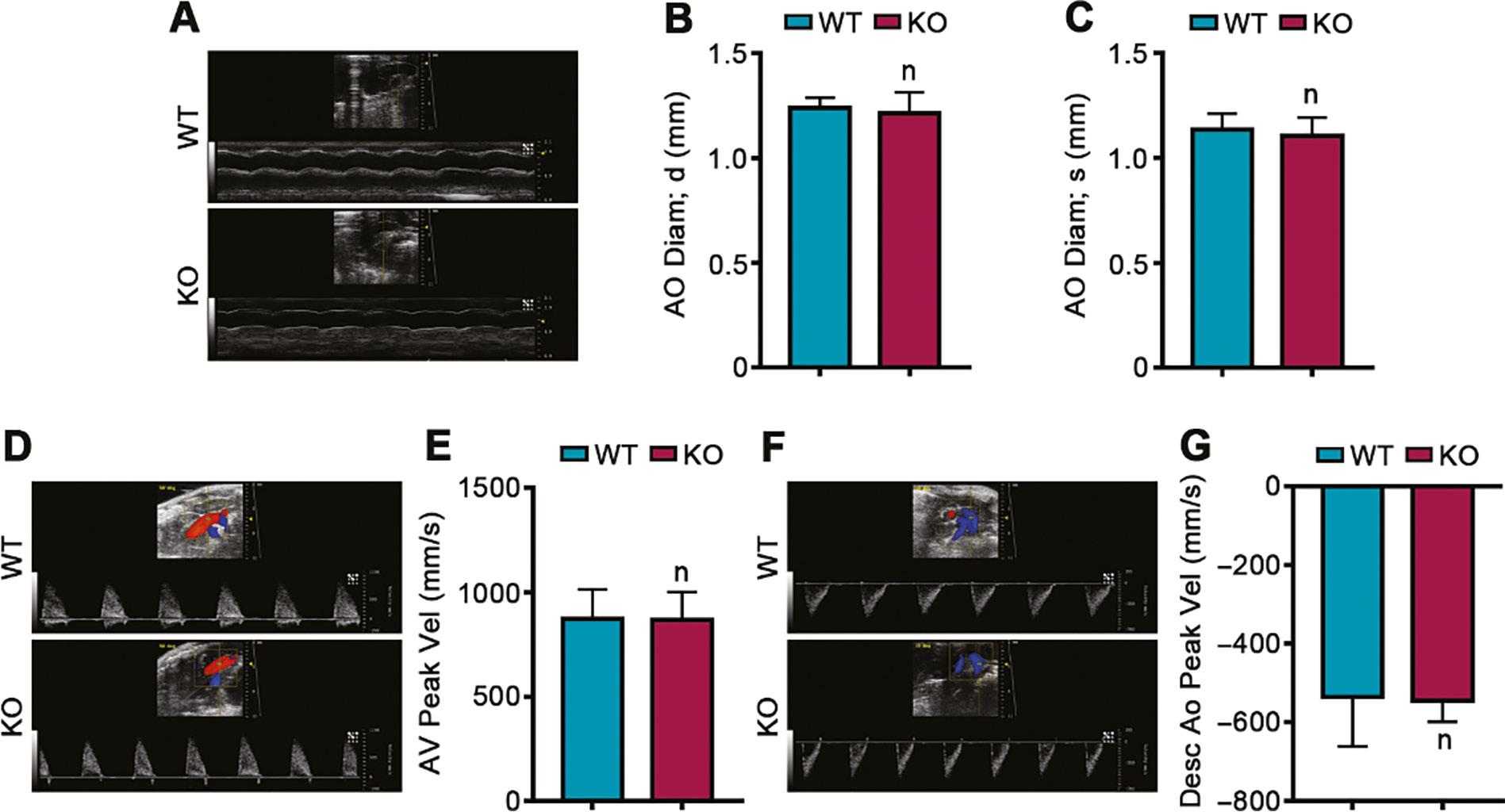
Effects of PEDF Deficiency on Vascular Hemodynamics During Cardiac Development.
A. Ultrasound images of Ao Diam measurement. B. Ao Diam at the end of diastole (n=4). C. Ao Diam at the end of systole (n=4). D. Ultrasound images of AV Peak Vel measurement. E. AV Peak Vel (n=4). F. Ultrasound images of Desc Ao Peak Vel measurement. G. Desc Ao Peak Vel (n=4). Ao Diam, aorta diameter; AV Peak Vel, peak velocity in aortic valve; Desc Ao Vel, peak velocity in descending aorta. Data are expressed as mean ± SD.
These results confirmed that PEDF deficiency during cardiac development does not significantly affect vascular hemodynamics.
Discussion
In this study, we clarified the expression profiles and functions of cardiac PEDF during cardiac development, and confirmed that cardiac PEDF expression significantly decreases after birth yet remains high, and is not involved in the developmental process of the heart. Therefore, PEDF deficiency during cardiac development does not have significant effects on cardiac structure, cardiac function or vascular hemodynamics. The contribution of this study is that it excludes effects of cardiac PEDF on cardiac development, thus contributing to understanding of the functions of PEDF in acquired heart disease.
Previous studies have demonstrated that PEDF is an important cardioprotective factor with significant protective effects in a variety of diseases, including MI and I/R [18–20]. Our analysis of several published datasets indicated that cardiac PEDF expression is elevated in a variety of heart diseases. This finding suggests that cardiac PEDF may exhibit compensatory elevated expression in early stages of various heart diseases, thereby resisting disease progression. In analysis of the GSE775 dataset, cardiac PEDF expression was sharply elevated at 1 week after MI but had largely normalized 8 weeks after MI, thereby reflecting the characteristic changes in cardiac PEDF expression from the compensatory elevation phase to the decompensated decline phase; this change may show a strong correlation with the stage of disease progression.
Although liver and adipose tissues are known to have a high PEDF expression, the level of PEDF expression in circulatory system tissues, including the heart and aorta, was previously unclear [13, 14]. Our analysis of PEDF expression in multiple tissues confirmed that PEDF expression levels are significantly higher in the heart and aorta than the liver. Our findings provide foundational evidence that PEDF is an important cardioprotective factor and aid in understanding of why PEDF is so important in cardiovascular disease.
Our results described changes in cardiac PEDF before and after birth in mice, and confirmed that cardiac PEDF expression decreases dramatically within 30 days after birth and is maintained until at least 60 days postnatally. The dynamic changes in cardiac PEDF expression suggest that, although PEDF does not significantly affect cardiac structure and function during cardiac development, it may be involved in specific physiological processes. Several significant alterations in physiological activities have been observed in mice before and after birth, such as cardiac energy metabolism patterns and cardiomyocyte proliferation [29–32]. Whether cardiac PEDF expression is involved in the alterations in related physiological activities remains unclear and deserves further in-depth study.
We emphasize that many previous studies have focused on the roles and mechanisms of PEDF in CVDs [33]. These studies have demonstrated that PEDF has significant protective effects in many CVDs, particularly in ischemic heart disease, through antioxidant, anti-inflammatory, anti-apoptosis and other functions [33]. In terms of mechanism, the known receptors of PEDF, including adipose triglyceride lipase (ATGL) and phospholipase A2 (PLA2), play key roles in PEDF’s protective effects regarding cardiovascular function [33]. Whereas previous studies have focused on the role of PEDF in pathological processes after the heart has matured, our study instead focused on the heart development process under physiological conditions during the heart development stage. Therefore, although our study confirmed that PEDF deficiency during cardiac development does not significantly affect cardiac structure, cardiac function and vascular hemodynamics, this feature is limited primarily to the first 8 weeks of life in mice. Whether corresponding alterations might occur again after a longer period of time remains unclear. Because PEDF is known to be an important regulator of lipid metabolism and a cardioprotective factor, and cardiac metabolic patterns are significantly altered before and after birth, whether the role of PEDF in regulating metabolism in the heart manifests after longer periods of time remains to be investigated [18, 34–36].
Conclusion
The expression of cardiac PEDF significantly decreases after birth, and it is accompanied by changes in subcellular localization characteristics, yet remains higher than that in the liver. Nevertheless, cardiac PEDF has no effects on cardiac structure, cardiac function and vascular hemodynamics during cardiac development.