Introduction
T1 mapping using cardiovascular magnetic resonance (CMR) introduces novel techniques for myocardial tissue characterization to detect and quantify disease processes occurring at the microscopic level. Rather than relying on nonspecific functional measures, T1 mapping focuses on intrinsic changes of myocardial composition that advances understanding about specific disease pathways. These changes in myocardial tissue composition inform diagnosis and prognosis.
T1 mapping encompasses two key parameters: native (i.e., precontrast) T1 and extracellular volume fraction (ECV) derived from additional postcontrast T1 and blood T1 measurements. Native T1 can reflect myocardial changes related to disease affecting the entire myocardium, whether intracellular or extracellular [1]. In contrast, ECV is more specific and mostly reflects myocardial changes limited to the extracellular interstitial space which includes the vascular compartment [1]. These two parameters appear to be robust for diagnosing specific disease processes [2]. These advances introduce new tools to detect focal and diffuse derangements in myocardial structure occurring in cardiac disease that can be otherwise difficult to detect [3–6].
Optimal diagnosis and prognosis promises optimal treatment whereby the patient's therapy can be matched to the underlying disease process. Indeed, this important principle of myocardial tissue composition-guided therapy has already been demonstrated with other myocardial CMR parameters [i.e., T2*(star)] whereby CMR guided care improved disease detection/severity measurement culminating in markedly improved patient outcomes [7]. It appears likely that T1 and ECV mapping will show similar impact in patient care. This review focuses on the emerging key principles involving the clinical application of T1 mapping.
Native T1, ECV, and Parametric Mapping
T1 is physical property of matter. The term “T1” refers to the exponentiated time constant representing the nonlinear recovery of longitudinal magnetization (spin-lattice relaxation) after a radiofrequency pulse. T1 is expressed in units of time (e.g., msec) and varies with temperature and chemical composition. Thus the accumulation of gadolinium based contrast agent, iron or glycosphingolipid can lower T1 in myocardium in proportion to severity. Practically, T1 is estimated by fitting the changes in signal intensity in T1 weighted images acquired at various time points following an inversion pulse. Modified Look Locker inversion (MOLLI) [8] is the most studied pulse sequence for myocardial T1 measurement, but other sequences also measure myocardial T1 [9–13]. Their relative merits and feasibility for clinical implementation remain areas of active investigation [14]. MOLLI uses a 3 parameter model to describe signal intensity (SI) as a function of exponentiated time after a 180 degree radiofrequency inversion pulse (TI):

where T1=T1*((B/A)−1) [8, 15, 16].
If myocardial T1 is additionally measured post gadolinium contrast (Gd), typically between 10 and 20 min after 0.1–0.2 mmol/kg bolus, Gd concentrations in the plasma and interstitium are in equilibrium because renal clearance is slow relative to its dispersion through the body. The uptake of Gd in the myocardium relative to plasma is a direct measure of the interstitial space with minimal dependence of gadolinium concentration since Gd contrast is extracellular [17]. ECV exploits this characteristic and is defined as:

where λ=[ΔR1myocardium]/[ΔR1bloodpool] pre and post gadolinium contrast (where R1=1/T1) [1].
The term “mapping” refers to the measurement of native T1 or ECV on a pixelwise basis. Mapping requires coregistration of component images from which the data are fit to generate the parameter estimates. Mapping permits visualization and spatial localization of T1 (Figure 1) or ECV (Figure 2). The signal intensity of regions of interest drawn on a T1 or ECV map by a reader yields the quantitative value of T1 or ECV. Color coding with look-up tables permits rapid qualitative interpretation based on visual inspection of the map.
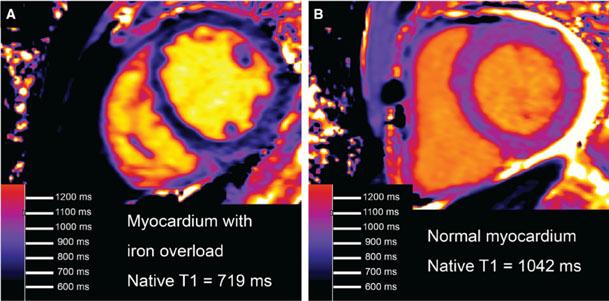
Native T1 in Human Myocardium Decreases with Excess Iron Content [18].
Panel A shows native T1 map shown from a patient with hemochromatosis and iron overload, demonstrating a remarkably low myocardial T1. In comparison to a patient with normal myocardial T1 who also happened to have a small pericardial effusion (panel B), panel A provides an image that is immediately recognizable as abnormal.
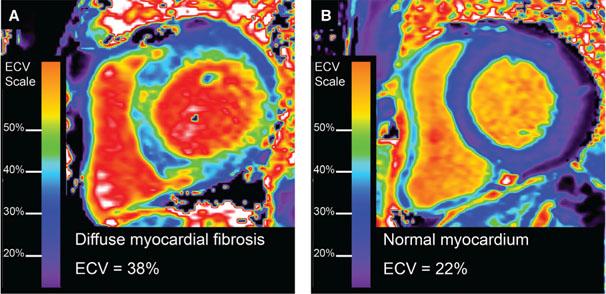
ECV mapping to depict and quantify diffuse myocardial fibrosis.
Examples of an ECV map quantifying the spatial distribution and extent of mostly diffuse fibrosis in a patient with nonischemic cardiomyopathy (Panel A). The image contrasts significantly with a patient without myocardial fibrosis with normal ECV measures (Panel B).
Even though T1 mapping has limited spatial resolution, cellular and molecular changes occurring within each voxel can affect the aggregate T1 signal rendering them quantifiable. The estimated T1-based parameters quantified on a T1 or ECV map demonstrate the spatial localization of these changes whereby each pixel expresses the quantitative value of that parameter. This quantitation permits detection of diffuse disease even if it is not directly visible (Figures 1 and 2).
These innovations are important because disease severity is quantified, and its spatial extent and distribution are also demonstrated on the map. Thus, myocardial disease can be rapidly detected regardless of whether focal or diffuse. Non quantitative “weighted” images, e.g., late gadolinium enhancement (LGE), are not robust for detecting diffuse disease and are not validated for this purpose [19]. LGE images are expressed in arbitrary units and can only detect spatial variation in myocardial fibrosis, e.g., “hot spots” relative to the most normal appearing myocardium which may not be normal at all. LGE cannot quantify the extent of diffuse interstitial expansion. Historically, diffuse myocardial disease has been difficult to measure or even appreciate noninvasively.
Pitfalls in T1 and ECV Mapping
T1 and ECV mapping demand more technical complexity for accurate image generation than T1 weighted imaging. It is imperative that the CMR practitioner be intimately familiar with several potential problems in image acquisition. Since T1 measures are sensitive to scanner characteristics and specific parameters related to sampling of T1 recovery, normative data from healthy volunteers should be generated for each scanner with minimal adjustment in the chosen T1 mapping pulse sequences thereafter; locking the T1 mapping protocols is recommended [1]. For the mathematical fitting of the T1 data, phase sensitive reconstruction [20] improves fitting of the data by removing a degree of freedom related to polarity and associated phase of the magnetization [i.e., SI=A−B·e(−TI/T1*) and the absolute value signs which previously introduced a degree of freedom are no longer present].
Creating the pixelwise map of T1 or ECV requires coregistration of all of the component images. Breath holding is therefore recommended to minimize misregistration for T1 and ECV mapping [1]. Motion correction improves coregistration of the component images from which the pixelwise maps are derived because breath holding does not always remove all respiratory motion [21]. Error maps [22] depict subsequent problems with data fitting. These latter images can alert the CMR reader to technological problems and/or associated artifact which may necessitate repeat measurements. These innovations improve the robustness of the technique, and they can be extended to ECV mapping [22]. In general, temporal and spatial resolution both must be sufficiently high to avoid artifact related to motion. Most protocols involve parallel imaging factors of 2 to maintain sufficiently high spatial and temporal resolution.
Partial volume averaging can corrupt myocardial T1 measures. The tissue from which T1 estimates are measured should be orthogonal to the imaging plane given the image slice thickness of 6–8 mm; obliquity and misregistration will inflate partial volume errors related to limited spatial resolution. Partial volume effects can be visualized as bands around the edges of the myocardium on T1 and ECV maps that depict pixels straddling the border between tissues (Figures 1 and 2). Partial volume error limits sampling of myocardial disease by T1 and/or ECV mapping to the mid myocardium since subendocardial and subepicardial pixels are “contaminated” by partial volume effects.
Off resonance can bias T1 measures significantly and represents a barrier to standardization between CMR sites, so optimal shimming is recommended to minimize off resonance [1]. These technical pitfalls are discussed in more detail in the consensus statement on myocardial T1 mapping and extracellular volume quantification from the Society for Cardiovascular Magnetic Resonance (SCMR) and CMR Working Group of the European Society of Cardiology [1].
Native T1 for Diagnosing Disease
Case control studies indicate that intracellular accumulation of iron in myocardial siderosis (iron overload) [18] or intracellular accumulation of glycosphingolipid in Anderson Fabry disease [23–25] lower native T1 in a diffuse fashion throughout the myocardium (Figure 1). Clinically, siderosis can present as a dilated cardiomyopathy phenotype whereas Anderson Fabry disease presents as a left ventricular hypertrophy phenotype. Neither of these two morphologic phenotypes (i.e., dilated cardiomyopathy or left ventricular hypertrophy) is sufficiently specific to inform the clinician of the underlying disease process. Thus, T1 measures add diagnostic specificity to these two morphologic phenotypes.
Since native T1 has the advantage of not requiring gadolinium based contrast, native T1 can be implemented as a rapid screening tool for Fabry's disease or iron overload. For example, the TIC-TOC study demonstrated the clinical and economic advantages of an ultrafast magnetic resonance imaging protocol for detecting myocardial siderosis [26]. This study scanned 6 participants per hour for two 12-h days, reducing scanning costs by a factor of ≈4. Native T1 mapping analysis took minimal training, required <1 minute, and was reliable.
Native T1 can also decrease in highly focal myocardial disease (i.e., not diffuse) such as the core of an acute myocardial infarction. [27, 28]. Shorter native T1 in the core of an infarct typically indicates more severe disease including microvascular obstruction and greater risks of adverse outcomes [29]. Native T1 can also depict the area at risk that occurs with acute coronary artery occlusion [30, 31].
Native T1 can increase in other conditions. These include myocardial fibrosis [32], amyloidosis [33–36] acute myocarditis [30, 37–42], acute myocardial infarction [16, 27, 28], vasodilation [43], and hypertrophic cardiomyopathy [44–46]. The origins of increased native T1 likely reflect increased water content (edema) in these conditions. The excess water in these diverse conditions may challenge the concept of what constitutes myocardial “edema” in the setting of diffuse disease. Native T1 in the setting of cardiac amyloidosis becomes especially high, a feature which can assist in distinguishing amyloidosis from fibrosis [33, 34, 36, 47] and also assist in prognosis [34].
Since so many disease pathways can alter native myocardial T1, the clinical context must be known to inform the clinician about the nature and extent of disease, like many valuable parameters in clinical medicine. Therefore, it is entirely expected that increased native generally T1 lacks specificity. Native T1 is most diagnostic when it is significantly decreased diffusely reflecting either Anderson Fabry disease or iron overload. When the native T1 is severely elevated, one must consider amyloidosis, especially in the setting of left ventricular hypertrophy and other suggestive ancillary findings. Otherwise, native T1 derangements can alert the clinician to myocardial disease such as fibrosis or edema, depending on the clinical context spanning the range from ambulatory patients to hospitalized patients post cardiac arrest.
Current recommendations advise each center to establish normal reference ranges since many scanner related factors and pulse sequence related factors can influence resultant native T1 measures [1]. Care must be taken to optimize the scanning protocol and then avoid subsequent changes in parameters to avoid confounding native T1 measurements.
ECV for Diagnosing Intrinsic Myocardial Disease
Myocardial fibrosis reflects dysregulation of collagen homeostasis. It occurs in a spectrum from mild to severe where excess collagen (concentration) accumulates in the myocardial interstitium from excess production and/or decreased catabolism [48]. Since gadolinium contrast agents are extracellular, ECV measures interstitial expansion occurring with fibrosis, amyloidosis, vasodilation, or interstitial edema [3, 4, 49]. Similar to native T1, these conditions can generally be differentiated if the clinical context is known.
ECV dichotomizes the myocardium into its primarily cardiomyocyte compartment and predominantly interstitial compartment (including the myocardial vasculature) [1]. ECV simply quantifies the interstitial uptake of gadolinium contrast agents relative to the plasma (Figure 2). Accordingly, histologic validation data overall show best agreement with ECV compared to other T1 metrics based on R2 values (i.e., the proportion of variation in a variable explained by another variable) [50, 51]. Specifically, ECV is superior to native [17, 52–55] or post contrast T1 [17, 52, 53, 56] in terms of agreement with collagen volume fraction. ECV is also superior to native T1 [57] or post contrast T1 [57] for predicting outcomes in large cohorts. Despite the potential confounding effects of capillary rarefaction which occurs in myocardial fibrosis [58] (since myocardial gadolinium contrast uptake includes the myocardial vasculature), most validation studies report high R2 values ≥0.6 when compared against the collagen volume fraction [17, 52–56, 59, 60].
ECV quantification of interstitial expansion represents a powerful tool to investigate myocardial remodeling, especially when combined with ancillary clinical data. While myocardial fibrosis may follow myocyte loss due to various types of injury, it also may occur with primary fibroblast activation [19]. The positive correlation between myocardial fibrosis (whether by ECV or histology) and left ventricular mass suggests significant prevalence of primary fibroblast activation since myocyte loss alone would decrease left ventricular mass [51]. This information may be relevant when appraising potential therapeutic targets to reverse myocardial remodeling.
The heart is similar to the liver, lung, and kidney: excess collagen can culminate in profound organ dysfunction and associated vulnerability [61]. Interstitial protein accumulation therefore reflects intrinsic organ disease. One need only consider the clinical example of cardiac amyloidosis to observe how disastrous interstitial protein accumulation can be for cardiac function, leading to symptoms and dismal survival. Thankfully, myocardial fibrosis exhibits at least some degree of plasticity, and various interventions can reverse it in humans [62–66].
“Interstitial heart disease” can affect microvascular, mechanical and electrical function as well as myocyte energetics [67], reflecting cardiomyocyte-extracellular matrix interactions beyond the interstitium. These interactions include: (a) capillary rarefaction and perivascular fibrosis [58] that limit perfusion reserve [62, 68, 69]; (b) myocardial stiffening [70, 71] from increased amyloid proteins [72] or from titin and collagen expansion with increased cross-linking in MF that leads to systolic and diastolic dysfunction [48, 63, 65, 69] and increased filling pressures [70], (c) impaired electrical conduction from disarray in the collagen network architecture that predisposes to reentrant arrhythmia and sudden death; [72, 73] and d) likely impaired cardiomyocyte/mitochondrial energetics if interposing excess collagen isolates cardiomyocytes from capillaries in the setting of decreased perfusion reserve and myocardial stiffening [51]. These concepts are illustrated in Figure 3.
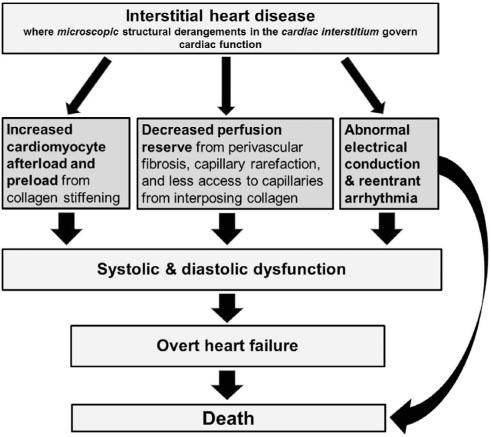
Interstitial heart disease represents interactions between excess collagen or amyloid proteins in the interstitium and other myocardial compartments. ECV quantifies the associated interstitial expansion. In the setting of myocardial fibrosis, it reflects excess collagen (mostly type I but also type III) secreted primarily by cardiac fibroblasts in the interstitium, a situation where synthesis predominates over degradation. Excess interstitial proteins adversely affect organ function and patient outcomes (modified from Schelbert EB, et al. J Am Heart Assoc. 2015;4:e002491).
Emerging data reveal that many cardiac insults culminate in myocardial fibrosis as a final common pathway, and the extent of fibrosis can vary across disease categories [2]. Similar to older studies limited to histopathology, myocardial fibrosis quantified by ECV can be observed in a variety of disease states including myocarditis [41, 74], rheumatologic disease [75–77], hypertension [78, 79], diabetes [80], obesity [81], heart failure regardless of ejection fraction [19, 52, 70, 82], and hypertrophic cardiomyopathy [59, 83, 84]. ECV does not appear to elevated in “Athlete's Heart,” suggesting that physiologic adaptation to exercise is primarily cellular (cardiomyocyte hypertrophy) rather than interstitial (myocardial fibrosis) [44].
Risk Stratification with T1 Mapping and ECV
Increased ECV reflects intrinsic myocardial disease. Indeed, the extent of myocardial fibrosis appears to govern vulnerability to adverse outcomes (death or heart failure) regardless of cause or disease category [19, 34]. The literature on ECV strongly suggests that it is a powerful tool to assess risk of adverse events and poor outcomes. In fact, ECV appears to be a more robust risk stratifier than left ventricular ejection fraction, the prognostic benchmark that governs so many clinical decisions in cardiology. ECV also appears to be a stronger risk stratifier than late gadolinium enhancement [19, 85].
ECV can measure myocardial fibrosis reasonably well despite the manipulation of myocardial tissue that occurs during histologic processing prior to microscopy that can alter the original in vivo interstitial space between cells. This alteration can potentially perturb the correlation between ECV and the collagen volume fraction. In addition, the distribution of collagen can vary spatially in the myocardium which also can alter ECV-collagen correlations. Diffuse myocardial fibrosis may have a subendocardial predilection in ischemic heart disease [86] rendering its ultimate quantification challenging by ECV which preferentially samples the mid myocardium. Nonetheless, surveying the literature, it is important to note that despite these limitations:
Histologic validation studies of ECV measuring myocardial fibrosis according to R2 value. The R2 value describes the proportion of variation in collagen volume fraction explained by ECV.
R2 value | Publication |
---|---|
0.89 | Miller CA, Naish J, Bishop P, Coutts G, Clark D, Zhao S, Ray SG, Yonan N, Williams SG, Flett AS, Moon JC, Greiser A, Parker GJ, Schmitt M. Comprehensive validation of cardiovascular magnetic resonance techniques for the assessment of myocardial extracellular volume. Circ Cardiovasc Imaging. 2013;6:373–383 |
0.80 | Flett AS, Hayward MP, Ashworth MT, Hansen MS, Taylor AM, Elliott PM, McGregor C, Moon JC. Equilibrium contrast cardiovascular magnetic resonance for the measurement of diffuse myocardial fibrosis: preliminary validation in humans. Circulation. 2010;122:138–144 |
0.77 | Zeng M, Zhang N, He Y, Wen Z, Wang Z, Zhao Y, Greiser A, An J, Zhang T, Jing B, Zhang X, Fan Z, Li D. Histological validation of cardiac magnetic resonance T mapping for detecting diffuse myocardial fibrosis in diabetic rabbits. J Magn Reson Imaging. 2016 |
0.72 | Aus dem Siepen F, Buss SJ, Messroghli D, Andre F, Lossnitzer D, Seitz S, Keller M, Schnabel PA, Giannitsis E, Korosoglou G, Katus HA, Steen H. T1 mapping in dilated cardiomyopathy with cardiac magnetic resonance: quantification of diffuse myocardial fibrosis and comparison with endomyocardial biopsy. Eur Heart J Cardiovasc Imaging. 2014;16:210–216 |
0.69 | White SK, Sado DM, Fontana M, Banypersad SM, Maestrini V, Flett AS, Piechnik SK, Robson MD, Hausenloy DJ, Sheikh AM, Hawkins PN, Moon JC. T1 Mapping for Myocardial Extracellular Volume Measurement by CMR: Bolus Only Versus Primed Infusion Technique. JACC Cardiovasc Imaging. 2013;6:955–962 |
0.69 | Fontana M, White SK, Banypersad SM, Sado DM, Maestrini V, Flett AS, Piechnik SK, Neubauer S, Roberts N, Moon J. Comparison of T1 mapping techniques for ECV quantification. Histological validation and reproducibility of ShMOLLI versus multibreath-hold T1 quantification equilibrium contrast CMR. J Cardiovasc Magn Reson. 2012;14:88 |
0.61 | de Meester de Ravenstein C, Bouzin C, Lazam S, Boulif J, Amzulescu M, Melchior J, Pasquet A, Vancraeynest D, Pouleur AC, Vanoverschelde JL, Gerber BL. Histological Validation of measurement of diffuse interstitial myocardial fibrosis by myocardial extravascular volume fraction from Modified Look-Locker imaging (MOLLI) T1 mapping at 3 T. J Cardiovasc Magn Reson. 2015;17:48 |
0.56 | Inui K, Tachi M, Saito T, Kubota Y, Murai K, Kato K, Takano H, Amano Y, Asai K, Shimizu W. Superiority of the extracellular volume fraction over the myocardial T1 value for the assessment of myocardial fibrosis in patients with non-ischemic cardiomyopathy. Magn Reson Imaging. 2016 |
Native T1 and ECV mapping have each shown promise for risk stratification in cardiac amyloidosis [34].
T1 Mapping and ECV for Monitoring Therapeutic Response in Practice or Phase 2 Efficacy Trials
T1 and ECV mapping technology may act as a catalyst for therapeutic development for Phase 2 efficacy trials. Due to the ability to track specific disease processes such as intracellular diseases affecting the cardiomyocyte (e.g., iron overload, Anderson Fabry disease) and extracellular/interstitial disease processes (e.g., myocardial fibrosis and cardiac amyloidosis) affecting the myocardial interstitium, investigators and clinicians can now enjoy robust, reproducible techniques that quantify the extent of myocardial disease. Rather than relying on nonspecific measures like left ventricular shape or function or natriuretic peptide levels, all of which can be influenced by preload, afterload, and volume status, investigators and clinicians can now probe how the myocardium in an individual may respond to proposed treatment. This issue is enormously important given the aging population and the epi-demic of heart failure where therapeutic progress has been slow [95–97]. In fact, whether the heart is the primary derangement in heart failure with preserved ejection fraction (“HFpEF”) is actively being debated. T1 and ECV mapping can now provide clear evidence of therapeutic response specifically located in the myocardium. Novel therapies targeting the interstitium are in development.
Conclusion
T1 and ECV mapping foster precision medicine and personalized care. Since T1 and ECV mapping elucidate specific disease pathways affecting myocardium, they promise to improve patient outcomes through targeted therapy. They are clinically robust and can easily be integrated into clinical scanning routines. Data thus far are promising, but further investigation is required to capitalize on the remarkable opportunities that T1 mapping and ECV introduce. These opportunities include improved understanding of disease mechanisms, therapeutic response to treatment, pharmacologic development, and optimizing therapeutic regimens through image guided care.