Abbreviations
CL: cathodoluminescence; DLs: tectonic deformation lamellae; EBSD: electron backscatter diffraction; EDS: energy-dispersive x-ray spectroscopy; EPI: epi-illumination microscopy; FFT: fast-Fourier transform; FIB: focused ion beam milling; GPa: gigapascal; OPT: optical microscopy; PDFs: planar deformation features; PFs: planar fractures; SEM: scanning electron microscopy; STEM: scanning transmission electron microscopy; TEM: transmission electron microscopy.
Introduction
In this study, we investigated quartz grains exposed to near-surface nuclear airbursts, in which the blast wave and fireball intersected the ground surface. We also compared shocked quartz grains from Arizona’s Meteor Crater, a relatively small (1.2-km-wide) impact structure. Our objective was to compare quartz grains exposed to pressures and temperatures associated with these two types of high-temperature, high-pressure events. We explored the hypothesis that low-altitude nuclear airbursts marked by relatively low pressures can produce shock fractures in quartz grains filled with amorphous silica. We also investigated whether these characteristic shock fractures in quartz grains formed similarly to those during crater-forming impacts, such as at Meteor Crater. Studies of crater-forming impacts and airbursts are crucial because they potentially have sudden, radical effects on the Earth’s environmental and biotic systems. However, most current studies have focused on ancient, large cratering events, such as that which occurred at the Cretaceous-Tertiary (KPg) boundary [1]. Relatively little is known about smaller, younger events, especially those caused by comets that may produce airbursts rather than large impact craters.
Previously, Eby et al. [2] and Lussier et al. [3] explored shocked quartz grains’ characteristics and formation mechanism resulting from the 1945 Trinity nuclear detonation at the Alamogordo Bombing Range, New Mexico. These studies revealed the presence of linear fractures that result from the high shock pressures of these detonations, leading Lussier et al. [3] to conclude that they may represent the initial deformational feature of quartz formed in a progression of increasing shock pressures. In another investigation related to the 1945 Hiroshima nuclear detonation, Wannier et al. [4] investigated glassy spherules but found that any shocked quartz grains that may have been present in the melt had been fully amorphized due to the extremely high temperatures.
Several laboratory experiments have investigated the shock-related transformation of quartz to amorphous silica. For example, in quartz grains experimentally shocked at 5 to 17.5 GPa, Fazio et al. [5] observed glass veins composed of amorphous silica extending across several microns in length and generally thicker than 50 nm. Wilk et al. [6] found amorphous silica in experimentally shocked rocks called shatter cones that formed at low shock pressures of 0.5–5 GPa. Shatter cones are considered to be a classic impact indicator. In addition, Carl et al. [7] conducted experiments demonstrating that extensive amorphization of quartz begins at ~10 GPa. Regarding the importance of amorphous silica in studies of shock metamorphism, French and Koeberl [8] wrote, “amorphous or ‘glassy’ phases … constitute another set of unique and distinctive criteria for the recognition of shock-metamorphosed rocks….” Similarly, Bohor et al. [9] wrote, “the formation of quartz glass within fractures … allows a definitive distinction … between these shock PDFs and the glass-free dislocation trails marking slow tectonic deformation.”
Even with these pioneering investigations, numerous questions remain about the formation of shock fractures and amorphous silica associated with nuclear airbursts. Is the formation process similar to that for planar fractures (PFs) and planar deformation features (PDFs) found in shocked quartz grains associated with cosmic impact craters? Are these features similar or different from tectonic lamellae in some deformed metamorphic rocks? In this contribution, we explore these and other questions.
Shock metamorphism in quartz
Previous studies of impact structures identified relatively low shock fractures in quartz and gave them various names, including shock extension fractures (SEFs) [10–13], shock fractures [14, 15], and vermicular (i.e., wormlike) microfractures [11, 13, 16]. Here, we adopt the term “shock fractures” to denote non-planar, shock-induced microfractures in quartz. They are often filled with amorphous silica, a term we use interchangeably with “glass.”
Multiple studies have reported different types of shock metamorphism observed in quartz, including planar deformation features (PDFs) [8, 9, 17–26], planar fractures (PFs) [8, 27], tectonic deformation lamellae (DLs) [8, 9, 14, 19, 21, 24, 28–31], and shock micro-fractures [26, 32–37]. Here, the term “lamellae” typically denotes parallel and planar stress features that form at high shock pressures in quartz. In contrast, the term “fractures” denotes typically open or glass-filled stress features that are sub-planar and sub-parallel. Table 1 compares some of the commonalities and differences among the types of shock features. Our analysis of previous studies (primarily French and Koeberl [8]) shows that shock fractures share 2 of 10 characteristics with PDFs, 4 of 10 with PFs, and 2 of 10 with DLs. Thus, shock fractures differ substantially from the other shock metamorphic features: PDFs, PFs, and DLs. The most important reported differences are that shock fractures are typically sub-planar, non-parallel, not crystallographically oriented, and form at lower shock pressures.
Characteristics of metamorphism of quartz.
Characteristics | Shock fractures | PDFs | PFs | DLs |
---|---|---|---|---|
Fractures rather than lamellae | Yes | No | Yes | No |
Features crystallographically controlled | No | Yes | Yes | Sometimes |
Multiple oriented sets of fractures/lamellae | Weakly oriented | 1–6+ | 1–6+ | 1–2 |
Planar fractures/lamellae | Sub-planar | Planar | Planar | Sub-planar |
Parallel fractures/lamellae | Sub-parallel | Parallel | Parallel | Sub-parallel |
Thickness of fractures/lamellae | nm to μms | Usually ≤1 μm | Usually ≥3 μm | Usually ≥2 μm |
Features filled with amorphous silica | Often | Often | Sometimes | Never |
Spacing between fractures/lamellae | nm to μms | Usually <1 μm | Usually >20 μm | Usually ≥5 μm |
Estimated formation pressure (Gpa) | ≥3 | ~10–25 | <10 | <1 |
Estimated formation speed | <1 sec | <1 sec | <1 sec | Very slow |
Shared features: | 2 of 10 | 4 of 10 | 2 of 10 |
Note. Shock micro-fractures investigated in this study share 2 of 10 characteristics with planar deformation features (PDFs), 4 of 10 characteristics with planar fractures (PFs), and 2 of 10 with tectonic deformation lamellae (DLs). The green shading represents features in common with shock fractures in our study. Data are primarily derived from French and Koeberl [8].
Key analytical studies of shock fractures
Kieffer [32] performed analyses of shocked sandstone from Meteor Crater and concluded that impact-related microfractures began to form at 5.5 GPa (Table 2, adapted from Table 2 of Kieffer [32]). Later, Kieffer et al. [33] described quartz grains within sandstone from Meteor Crater that were weakly shocked at <10 GPa and displayed fractures with quartz that was transformed into amorphous silica. For moderately and strongly shocked rocks, they proposed a process called “jetting,” in which molten quartz was injected under pressure into shock-formed fractures in the grains.
Classification of shock stages for quartz.
Shock stage | Range, low (GPa) | Range, high (GPa) | Lithology |
---|---|---|---|
0 | ≤0.2 | 0.9 | Undeformed, porous sandstone |
1a | 0.9 | 3 | Compacted, porous, deformed sandstone |
1b | 3 | 5.5 | Compacted, non-porous, deformed sandstone |
2 | 5.5 | 13 | Dense sandstone with 3 to 10 wt% glass and <95% quartz |
3 | 13 | 39 | Dense sandstone with up to 20 wt% glass and 45 to 80 wt% quartz |
4 | >30 | – | Dense sandstone with 20 to 75 wt% glass and 15 to 45 wt% quartz |
5 | – | – | Vesicular rock with 80 to 100 wt% glass and up to 15% quartz |
Note. Based on a study of quartz-rich sandstone from Meteor Crater [32, 33]. The scale ranges from unshocked quartz at shock stage 0 to highly shocked quartz at shock stage 4 and melted quartz glass at shock stage 5. Shock-generated fractures with amorphous silica (glass) first appear at ~5.0 to 5.5 GPa, as green highlighting indicates. This classification is from Kowitz et al. [11], based on Table 2 of Kieffer [32, 33] and modified by others [38, 39].
Christie et al. [18] performed laboratory experiments on milled quartz cylinders by generating slow-strain conditions to produce glassy lamellae using a confining pressure of 1.5 GPa and a stress differential of up to 3.6 GPa. Their experiment attempted to replicate the features known to form in quartz grains during tectonic motion along fault planes. They reported the presence of deformation lamellae closely associated with amorphous silica at low pressures under laboratory conditions. Their experiment suggests that glass-filled lamellae may form in quartz at pressures as low as 1.5 GPa.
Importantly, Christie et al. [18] did not report amorphous silica associated with naturally-formed tectonic deformation lamellae in quartz [19], suggesting that their laboratory experiments did not replicate the processes that form natural tectonic lamellae. Co-author H.-R.W. has performed multiple analyses of tectonic lamellae and, notably, never observed amorphous silica associated with tectonic lamellae in quartz grains [40–44]. In addition, Houser et al. [45] described finding tectonically-formed, nano- to micro-scale amorphous silica particles and nanofilms along active fault planes, but they reported no quartz grains with fractures containing amorphous silica. Multiple studies have observed amorphous silica within fractures, but only in impact-related shocked quartz and not in tectonic deformation lamellae [9, 14, 19].
Laboratory experiments by Kowitz et al. [11, 15, 46] investigated the shock alteration of quartz grains when a steel plate was explosively driven into cylinders of quartz-rich sandstone at pressures of ~5, 7.5, 10, and 12.5 GPa (Figure 1). Visible shock fractures and amorphous silica (~1.6 wt%) first appeared at ~5 GPa [11]. Scanning electron microscopy (SEM) images reveal shock fractures called “sub-planar, intra-granular fractures [11].” This result is significant because most shocked material within small impact craters forms within this lower shock pressure range. The combined shock effects from studies by Kieffer [32, 33] and Kowitz et al. [11, 15, 46] are summarized in Table 2.
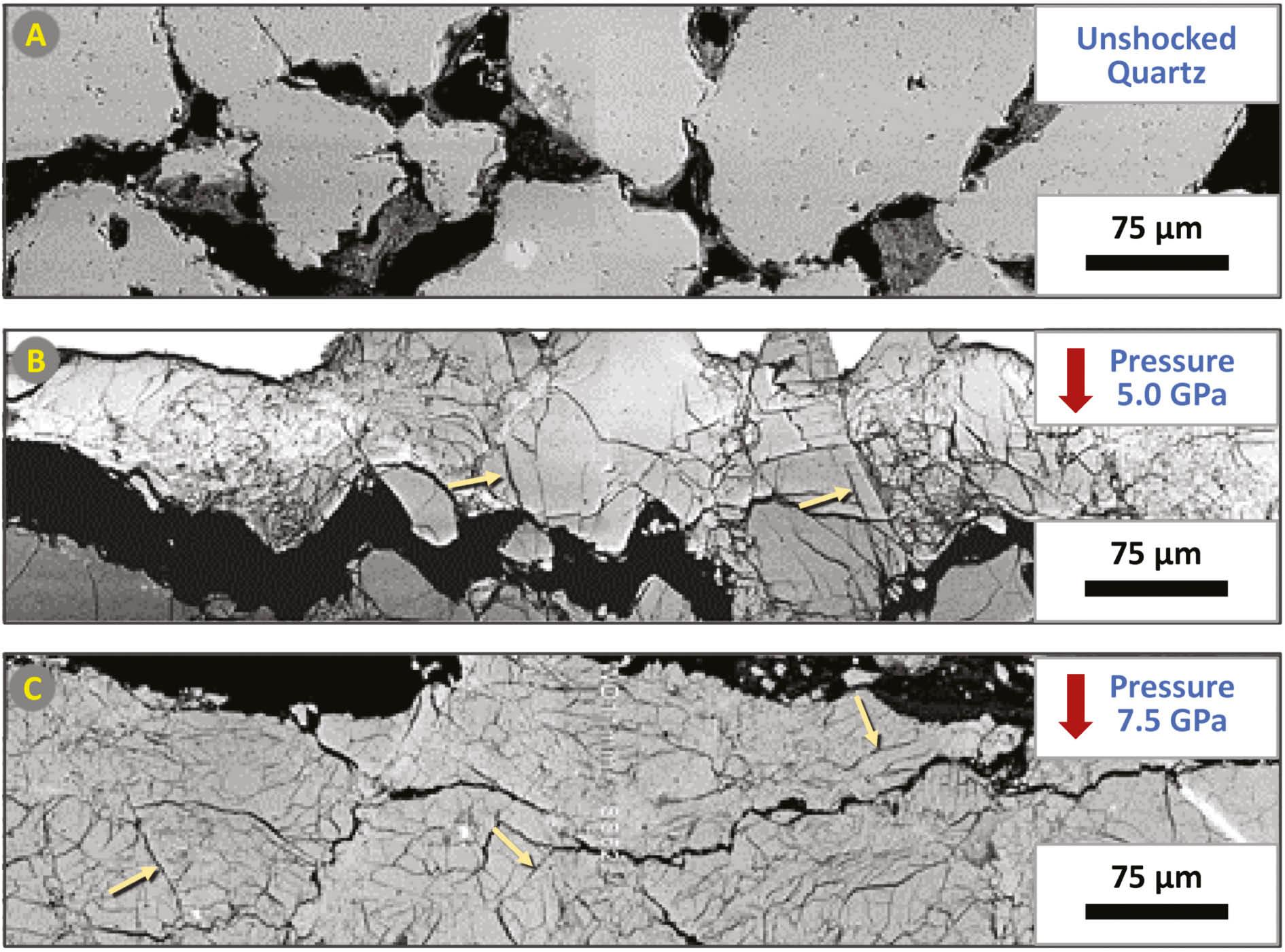
Low-shock fractures in quartz. SEM backscatter electron (BSE) images of polished, thin-sectioned grains from shock experiments by Kowitz et al. [11] showing (A) original unshocked quartz grains in porous sandstone; (B) grains with non-planar, intra-granular microfractures initially produced at 5 GPa; (C) grains shocked at 7.5 GPa. Red arrows mark the direction of the applied shock from the top of the images down; yellow arrows mark selected representative fractures. Adapted and cropped from Kowitz et al. [11]; used with permission.
The Trinity nuclear airburst has been recently estimated at 24.8 ± 2 kiloton (kt), up from previous estimates of 20–22 kt. The presence of various key minerals is indicative of the extreme pressures generated: ~8 to < 10 GPa for shocked quartz [2, 3]; ~7–10 GPa for shocked zircon [47]; <25–60 GPa for vesiculated feldspar [48]; >8 GPa based on the fractionation of zinc [49]; and 5–8 GPa based on quasi-crystalline minerals in trinitite [50]. These studies of the Trinity airburst are critical because they establish the high pressures typically necessary for producing shock metamorphism.
Sample locations
Meteor Crater, Arizona
This site, also known as the Barringer Crater, is a 1.2-km-wide hypervelocity impact feature located east of Flagstaff, Arizona [51]. The 180-m-deep crater is surrounded by an ejecta blanket that is elevated ~30 to 60 m above the local surface (Figure 2). The 50,000-year-old impact crater is estimated to have been produced by an approximately 50-m-wide bolide, now known as the Canyon Diablo meteorite [51]. The bedrock inside Meteor Crater contains shocked quartz with high-pressure planar deformation features (PDFs) [32, 51], but we limited our study to shock-fractured quartz grains embedded in samples of meltglass that had been ejected from the crater; we did not examine quartz grains embedded in sandstone or limestone (Appendix, Figure S1). The samples were collected in 1966 by Bunch [51] on the rim ~500 m north of the crater’s center at ~35.032206° N, 111.023988° W.
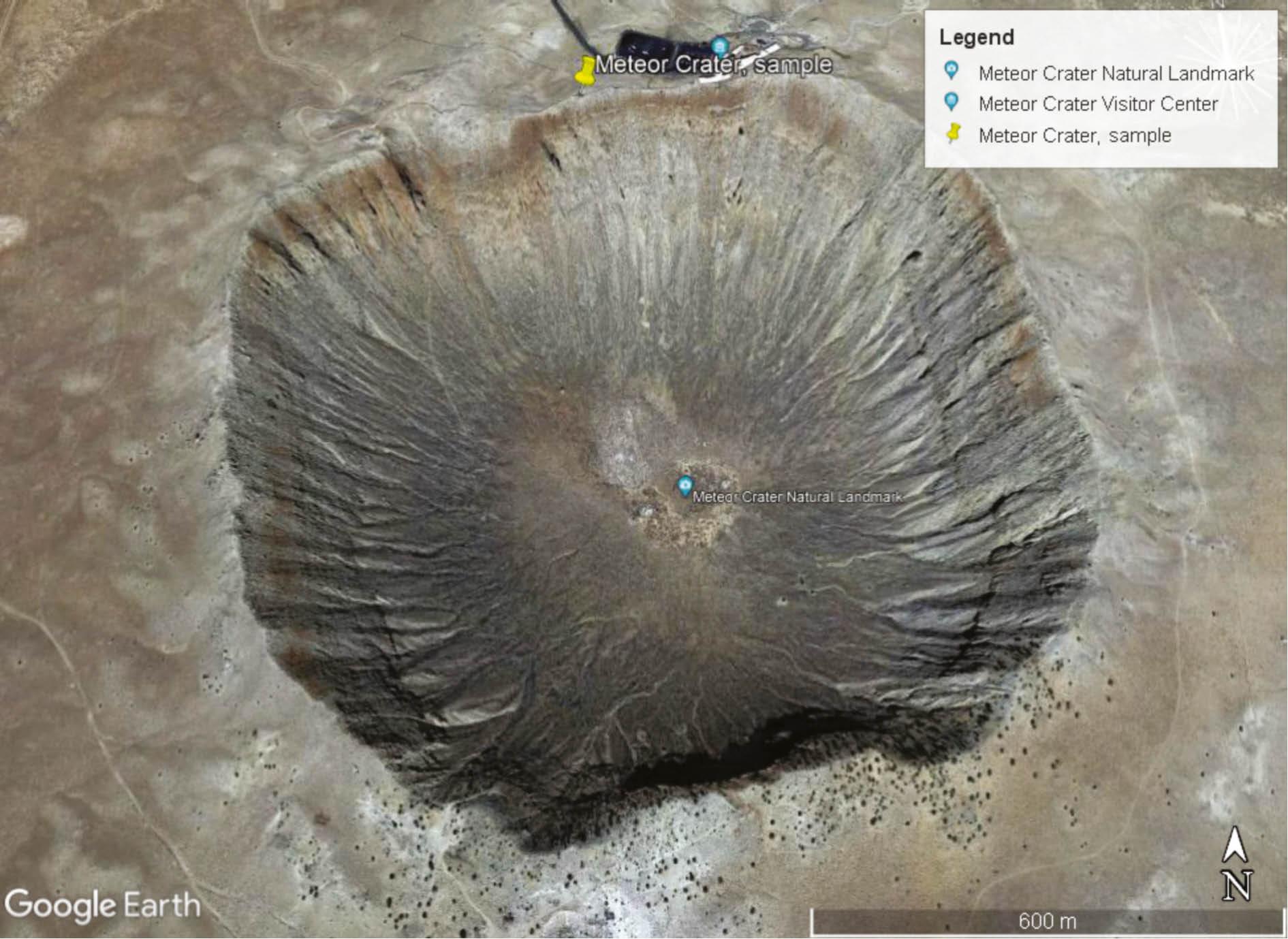
Meteor Crater, Arizona. 1.2-km-wide impact crater near Flagstaff in northern Arizona [51]. The 180-m-deep crater is surrounded by an ejecta blanket elevated ~30 to 60 m above the plateau. Source: “Meteor Crater” 35.032206° N, 111.023988° W. Google Earth. Imagery date: 2022. Yellow pin marks the location of sample analyzed. Accessed: 10/08/2022. Permissions: https://about.google/brand-resource-center/products-and-services/geo-guidelines/.
Russia, Joe-1 and Joe-4 nuclear tests, near-surface airbursts
The first Soviet nuclear bomb test, nicknamed “Joe 1” by the Americans, was conducted in 1949 in Kazakhstan (~50.590664° N, 77.847319° E). The ~20-kt nuclear test was detonated aerially on a 30-m-tall tower (Figure 3). “Joe 4” is the American nickname for a 400-kt Russian test that was detonated on a 30-m-tall tower at the same location in 1953. This study analyzed only fractured quartz grains in loose sediment and embedded in multi-mm-sized fragments of meltglass. A surface sediment sample was collected by Byron Ristvet on 9/1/2012 at ~100 meters from ground zero for both tests (Appendix, Figure S2). It could not be determined which nuclear test produced the sample that was collected and investigated.
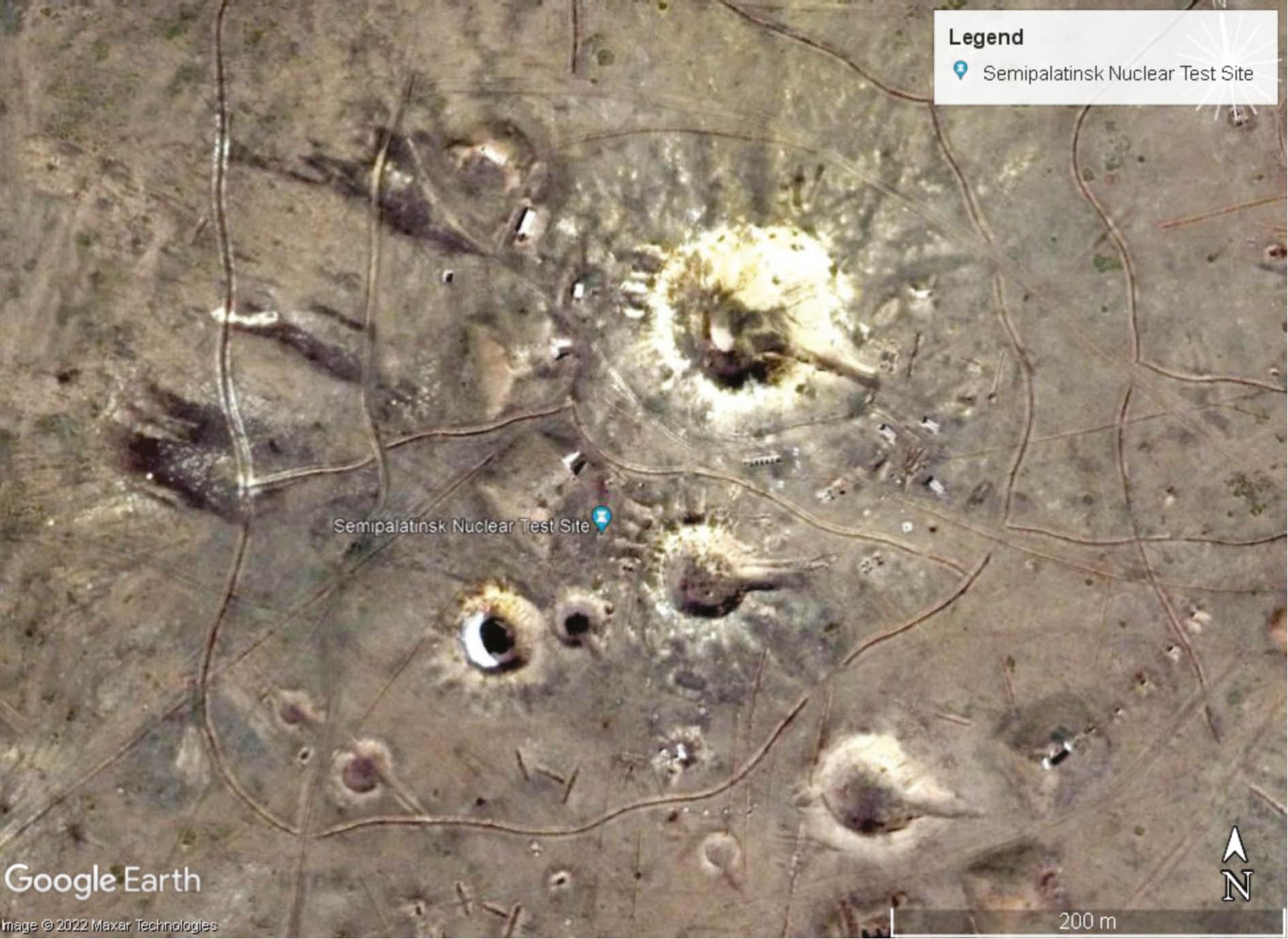
Semipalatinsk Nuclear Test Site, Kazakhstan. Photo of the general area for the detonations of Joe-1 and Joe-4 and other nuclear bomb craters. Source of base image: “Joe-1 test” 50.590664° N, 77.847319° E. Google Earth; Maxar Technologies. Imagery date: 2022. Accessed: 10/08/2022. Permissions: https://about.google/brand-resource-center/products-and-services/geo-guidelines/.
U.S., Trinity nuclear test, near-surface airburst
The Trinity nuclear bomb was detonated aerially in 1945 at the Alamogordo Bombing Range, New Mexico, on a tower at an altitude of 30 m [2] with an estimated energy of 24.8 kilotons (kt) of TNT equivalent [52]. The fireball was ~300 m wide at ~25 ms after detonation (Figure 4A). A blast zone of the ejected materials extended more than 400 m radially from ground zero [2]. The airburst formed a crater that was ~80 m in diameter [53] and ~1.4 m deep [54] (Figure 4B). This study analyzed only fractured quartz grains embedded in meltglass, called trinitite, which was collected by co-author R.E.H. on 9/30/2011 from the ground surface ~400 m north of ground zero (33.68100° N, 106.4756° W) (Appendix, Figure S3). R.E.H. also studied another sample (JIE) of loose quartz grains found on an anthill near ground zero, collected by Jim Eckles in 2003.
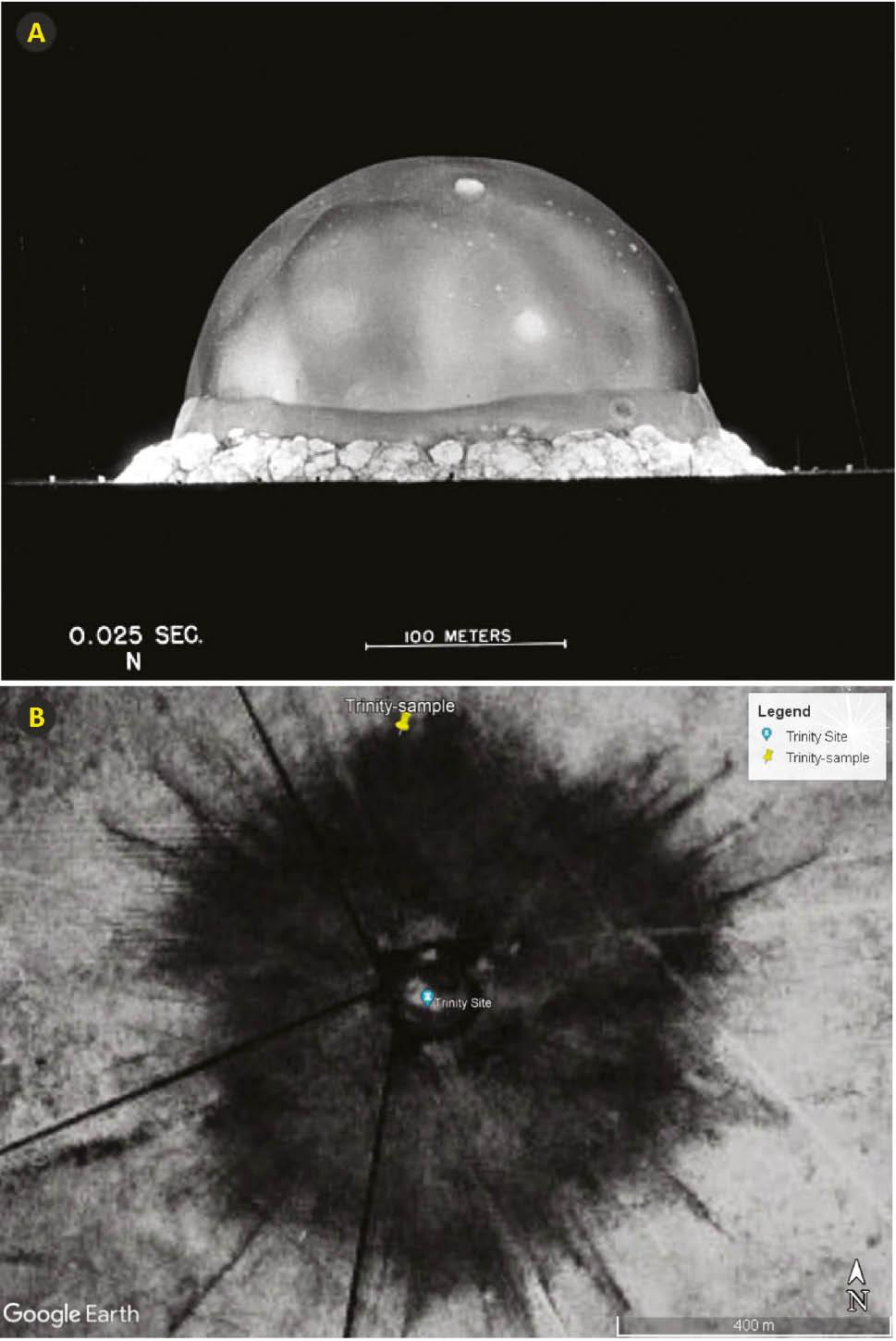
Trinity Test Site in New Mexico. (A) Trinity ~300-meter-wide nuclear fireball, taken 25 ms after 24.8-kt detonation. Photo date: July 16, 1945. Courtesy of US Govt. Defense Threat Reduction Agency. Source: http://www.nucleararchive.com/Photos/Trinity/image8.shtml This work is in the public domain. (B) Post-detonation photograph. The photo was taken in July 1945, approximately 28 hours after the blast. The dark color represents trinitite glass within a ~400-meter-radius blast zone, which was discontinuously covered with ejected dark trinitite glass. Note that dark streaks of material radiate from ground zero. Source: “Trinity,” 33.68100° N, 106.4756° W. Google Earth. Imagery accessed: 10/08/2022. Permissions: https://about.google/brand-resource-center/products-and-services/geo-guidelines/
Sampling and methodology
Samples were collected as described in the Appendix, Methods-Samples. Candidate grains were processed as described in the Appendix, Methods-Processing Steps. The Appendix also lists the locations of laboratories where analyses were performed. Selected grains were investigated using multiple standard analytical techniques and preparation methods, as described in Methods below and the Appendix, Methods-Analytical Techniques.
Results and discussion
We employed ten analytical techniques to investigate shock fractures containing amorphous silica, as follows:
Optical transmission microscopy (OPT)
Using this technique, we observed that >50% of the grains examined for each of the three sites displayed shock fractures. Representative optical and SEM-BSE images of quartz grains are shown in Figure 5. These images are comparable to those from the experimental study shown in Figure 1. Most displayed a single set of shock fractures, meaning all are oriented in approximately the same direction. However, a few grains display multiple sets oriented along different axes.
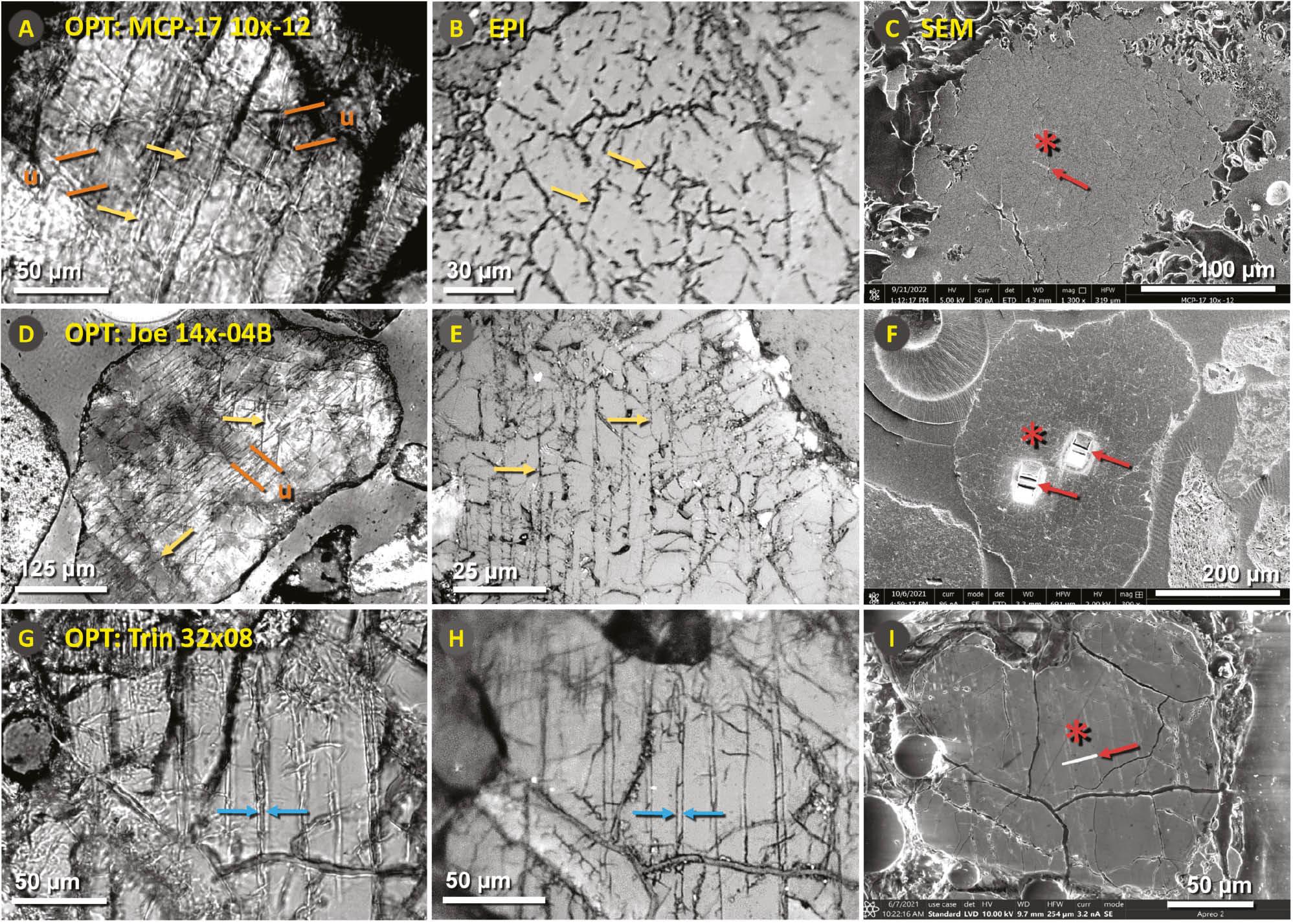
Images of fractures in quartz grains. Optical microscopy (OPT), left-hand panels A, D, G. Epi-illumination (EPI), middle panels B, E, H. Scanning electron microscopy (SEM-BSE), right-hand panels C, F, I. (A-C) Grains from Meteor Crater, Arizona. (D-F) Grains from the Russian Joe-1/4 nuclear test. (G-I) Grains from the Trinity nuclear test site. Optical images (left-hand column) were acquired under crossed polarizers rotated ~20° off maximum for better visibility. Yellow arrows indicate random representative shock fractures. Panels A and D show dark bands of undulose extinction between orange lines labeled “u.” The Trinity grain in panels G and H displays oriented pairs of shock fractures between blue arrows. Red arrows in panels C, F, and I (right-hand column) mark sites from which micron-sized slices of the quartz grain were removed using the focused ion beam (FIB) and then analyzed using bright-field TEM and TEM-EDS. The red asterisks in the right-hand column mark the locations of CL and SEM-EDS analyses.
Some grains with shock fractures display undulose extinction (Figure 5), in which waves of extinction are typically oriented perpendicular to the trend of the grain’s lamellae. Kowitz et al. [15] reported that the extinction of quartz grains is sharp in unshocked sandstone. In contrast, they noted that undulose extinction becomes apparent in sandstone shocked to 5 GPa, transitioning to weak but still prominent mosaicism (i.e., irregular patchwork extinction) (Figure 5).
Epi-illumination microscopy (EPI)
This analytical technique is particularly useful in viewing HF-etched quartz grains (Figure 5) that display previously hidden glass-filled fractures. Multiple studies [9, 14, 19, 21, 55, 56] have demonstrated the usefulness of performing analyses after etching quartz grains with HF. According to Gratz et al. [19], the HF-etching removes some amorphous silica filling the shock features, allowing for the “unambiguous visual distinction between glass-filled PDFs and glass-free tectonic deformation arrays in quartz.” Other techniques are necessary to identify and characterize the filled material as amorphous silica, a key indicator of shock metamorphism [9, 19].
In contrast, lamellae in tectonically-deformed grains are not visible in EPI as open fractures but may appear as shallow, closed depressions without filling material. Our investigations of six unshocked natural quartz grains and six tectonically-deformed quartz grains from non-impact layers reveal that none contain amorphous silica. See Figure 15 and 16.
Scanning electron microscopy (SEM-BSE)
Analyses using SEM-BSE revealed filled fractures in quartz grains that appeared mostly as linear features, although some were curvilinear. Other analyses are also necessary to identify and characterize the material filling the fractures.
Scanning transmission electron microscopy (STEM)
FIB locations of analyzed grains are shown in Figure 5. Using dark-field STEM, the 8- to 15-μm-wide foils display inter-fracture spacings ranging from ~250 nm to 3 μm (Figure 6). Nearly all shock fractures were observed to contain material that was shown to be amorphous silica discontinuously filling the fractures.
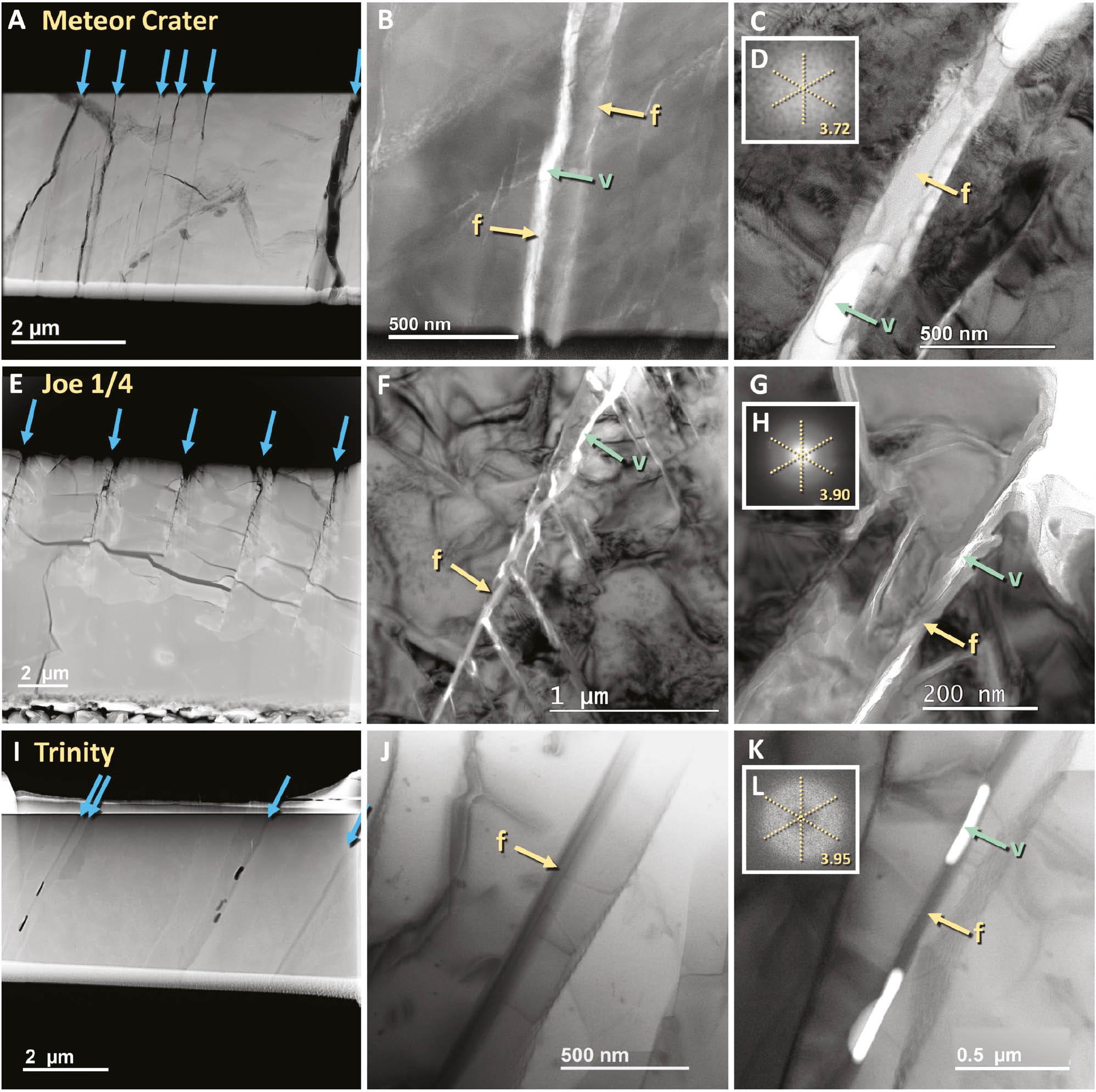
Images using STEM, TEM, and fast-Fourier transform (FFT). (A-D) Grain #10x-12 from Meteor Crater, Arizona. (E-H) Grain #14x-04 from the Russian Joe-1/4 nuclear test. (I-L) Grain #09x11 from Trinity meltglass. The blue arrows mark shock fractures (left-hand column) in these dark-field STEM images, in which the dark lines represent fractures, and the black areas represent voids. For bright-field TEM analyses (middle and right-hand columns), arrows labeled “f” mark material that discontinuously fills the shock fractures. Green arrows labeled “v” indicate voids that appear white in bright-field TEM mode rather than black as in dark-field STEM. Panels D, H, and L are FFTs. The diffuse halo and the d-spacings of its outer edge indicate that the filling of the fractures is amorphous silica. Halo d-spacings were measured along dashed yellow lines and averaged 3.72 Å in panel D, 3.90 Å in panel H, and 3.95 Å in panel L. The diameter of the bright-field TEM beam spot was ~0.5 μm. Insets of diffraction spectra were acquired at “f” in each corresponding bright-field TEM image.
Transmission electron microscopy (TEM)
Images acquired using bright-field TEM show sub-planar shock fractures containing thin bands of amorphous silica (Figure 6). We confirmed the amorphous state of the fill material using high-resolution bright-field TEM that can image individual atoms (Figure 7).
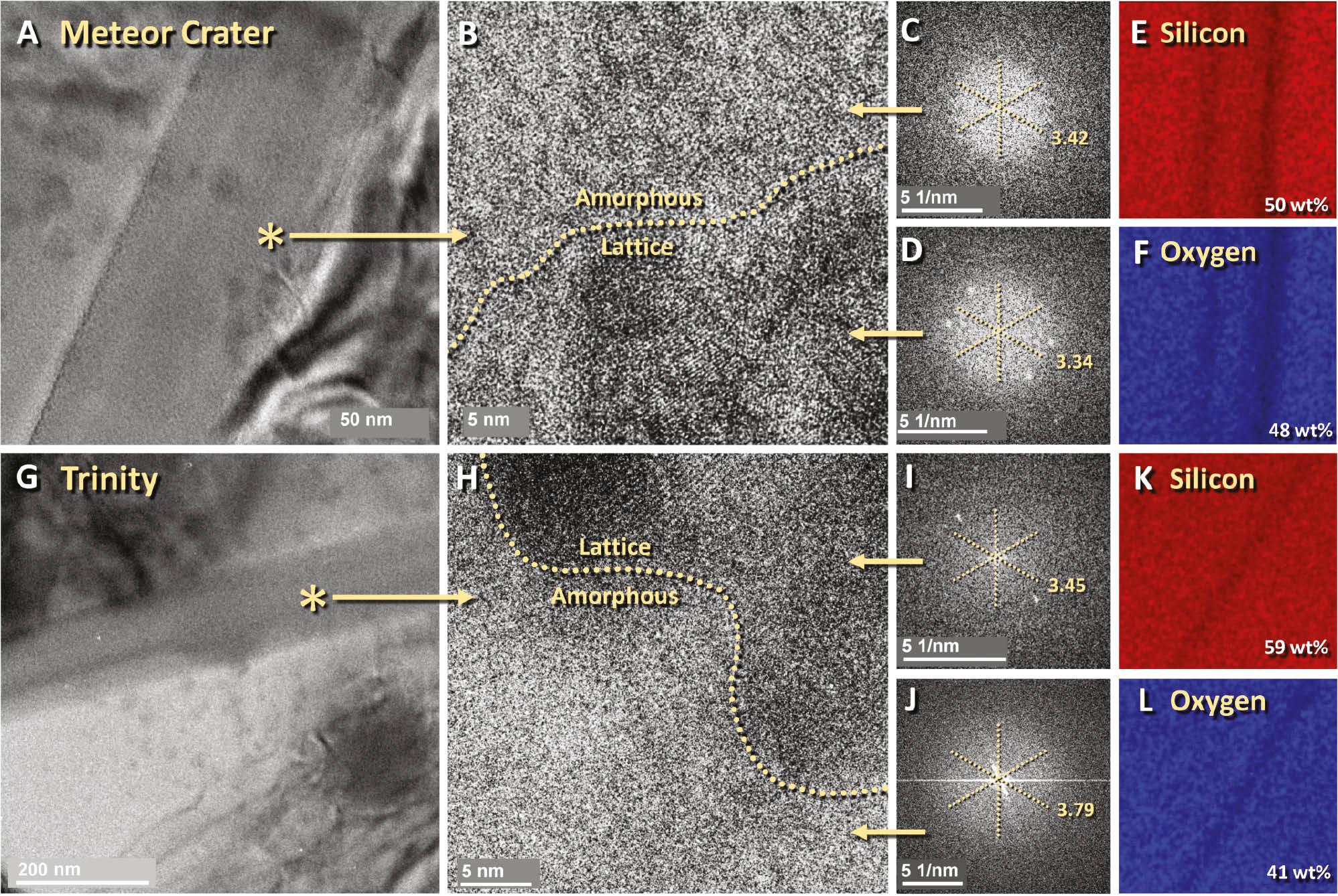
TEM images of quartz shock fractures filled with amorphous silica. (A-F) is from Meteor Crater (grain #09x-11); (G-L) is from Trinity (grain #09x11). (A) Bright-field TEM image of the region of interest. (B) A close-up bright-field TEM image exhibits the crystalline lattice below the dotted line and the amorphous silica above; the image was acquired at the asterisk in panel A. (C) Fast-Fourier transform (FFT) of the top part of panel B exhibits a diffuse halo indicative of amorphous silica with a d-spacing of 3.42 Å. (D) FFT of the bottom part of panel B exhibits diffraction spots with a halo indicative of a mix of crystalline lattice with amorphous silica. The halo measures 3.34 Å. (E-F) EDS panels show a composition of 98 wt% silica; the EDS spectra were acquired at the location of the asterisk in panel A. (G) Bright-field TEM image of the region of interest. (H) A close-up bright-field image exhibits the crystalline lattice above the dotted line and the amorphous silica below the line; the high-resolution TEM (HRTEM) image was acquired at the location of the asterisk in panel G. (I) FFT of the top part of panel H shows diffraction spots with a halo that measures 3.45 Å. (J) FFT of the bottom part of panel H displays a diffuse halo indicative of amorphous silica. The d-spacing of the amorphous halo is 3.79 Å. (K-L) EDS panels show a composition of 100 wt% silica; analyses were acquired at the location of the asterisk in panel G.
Numerous inclusions, also known as decorations or vesicles, are filled with glass or gases and are closely associated with shock fractures (Figure 6). Madden et al. [57] reported that multi-phase inclusions of glass, gases, and fluids are typical at Meteor Crater in sandstone lightly shocked at ≥5.5 to 13 GPa. In contrast, that study observed no multi-phase inclusions in samples formed at >13 GPa in shock stages 3 or 4, suggesting that the high shock pressures collapsed the inclusions [57]. Thus, the evidence suggests that these grains with shock fractures formed at low pressure of 5 to 13 GPa at shock stages 1 to 2. In contrast, unshocked tectonically-deformed quartz grains may display lines of bubbles, known as decorations, that form by the dissolution of quartz by water rather than by shock-related processes.
Fast-Fourier transform (FFT)
The areas of the grains from which the foils were extracted are shown in Figure 5. In this study, the FFT analyses commonly displayed crystalline structure in the quartz matrix away from the shock fractures, but most shock fractures displayed a diffuse halo or ring indicative of amorphous material [33, 58, 59], especially in the thin bands of glass along the shock fractures (Figures 6 and 7).
FFTs of the filling along these thin fractures display the diffuse halo-like patterns characteristic of amorphous material [33, 58, 59]. The halos have average d-spacings of ~3.72 Å for Meteor Crater, ~3.90 Å for Joe-1/4, and ~3.95 Å for Trinity (Figure 6). Other average halo d-spacings are shown in Figure 7. The mean value of 10 grains for the three sites is 3.60 Å with a range of 3.34 to 3.95 Å. Plots show typical halo d-spacings for each of the three sites that are somewhat lower than the reported halo d-spacing of 4.2 Å for quartz glass [60] (Figure 8).
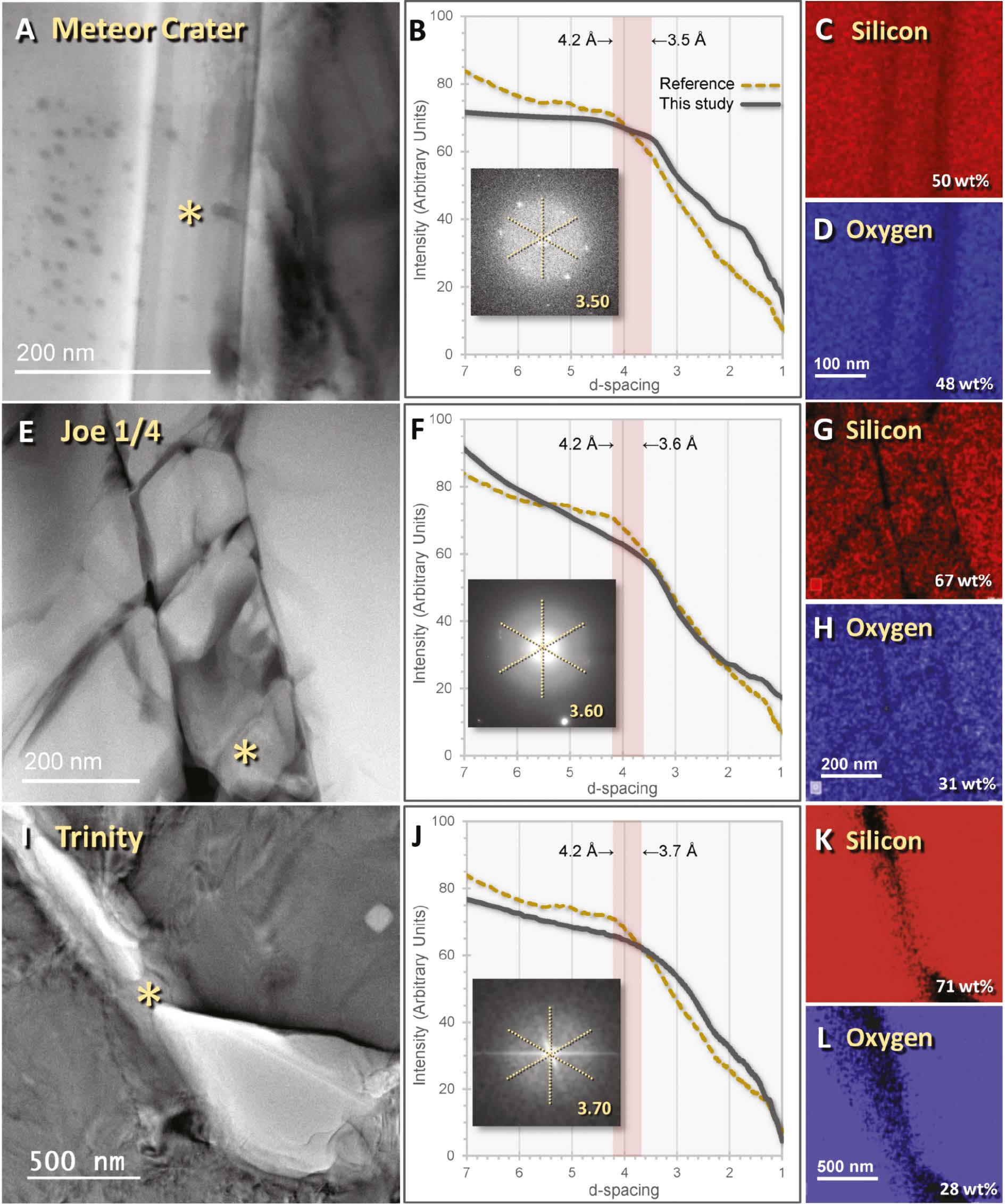
TEM images; FFT patterns and plots; EDS elemental maps. All images were acquired from FIB foils. (A-D) Grain #10x-12 from Meteor Crater, Arizona. (E-H) Grain #14x-04 from the Russian Joe-1/4 nuclear test. (I-L) Grain #30x08 from the Trinity JIE sediment sample. Bright-field TEM images (left-hand column) show the micron-sized areas analyzed; asterisks mark the locations used to generate the FFTs (middle column insets) and the EDS analyses (right-hand column). Panel I (Trinity) shows a glass-filled shock fracture intersecting a glass-filled vesicle. In the middle column, the graphs show intensities plotted against d-spacings generated from FFTs using the Profile function of Digital Micrograph, version 3.32.2403.0. Each grain in this study shows a decrease in slope at d-spacings ranging from 3.50 to 3.70 Å (black line), marking the edges of the diffuse halos shown in the FFT insets. The yellow dashed lines plot a reference profile of non-shocked amorphous silica (melted quartz) [60] with a slope change at 4.20 Å. The slopes of the yellow and black lines are similar, consistent with the presence of amorphous silica in the grains in this study. EDS analyses in right-hand panels confirm that the areas centered on the asterisks in the left-hand panels are predominantly silica and oxygen (range: 98–99 wt%).
Gleason et al. [61] conducted experiments on amorphous silica and noted that unshocked amorphous silica had a d-spacing of about 4.20 Å. In contrast, shock pressures ranging from 4.7 to 33.6 GPa transformed the quartz into amorphous silica that was permanently densified, causing the standard glass d-spacing to decrease within a range of 3.36 to 4.00 Å. Thus, in our study, the lower d-spacing values (mean = 3.62 Å) support an interpretation that amorphous silica from the three sites was shocked and densified at as low as 4.7 GPa.
TEM energy dispersive spectroscopy (TEM-EDS)
Energy-dispersive X-ray spectroscopy (EDS) is an analytical technique used to determine the elemental composition of materials. EDS analyses of multiple grains demonstrated that most of the material filling fractures is predominantly composed of silicon and oxygen (range: 98–99 wt%). Together with the diffuse rings exhibited in the FFT results (Figures 6–8), this finding confirms that the material filling the fractures is amorphous silica. On the other hand, this Si-rich material is inconsistent with being hydrated silica (opal, hyalite) that can precipitate into fractures because the filling lacks spherical micro-structures typically present in opal [62]. Furthermore, TEM-EDS analyses reveal insufficient levels of oxygen to account for the hydration of silica (opal, hyalite) [62]. Concentrations typically total ~66 wt% oxygen in opal and hyalite [62], compared with ~28 to 48 wt% for the glass in our samples. For EDS spectra and other details, see Appendix, Figures S4–S7.
Most material that fills the fractures is amorphous silica, but some fractures are intermittently filled with C, Al, Mg, Fe, or Ca. These represent secondary materials possibly injected into the fractures during their formation, precipitated later into the fractures, or introduced during the preparation and polishing of samples.
Cathodoluminescence (CL)
The areas of grains analyzed for CL are shown in Figure 5. Representative CL images are shown in Figures 9–11. Under CL, fractures filled with amorphous silica have been reported to be commonly non-luminescent, i.e., black [21, 59, 63], although some defect structures in amorphous silica have been reported to luminesce red [65]. Alternately, open fractures also appear black; therefore, TEM and TEM-EDS must be used to confirm the possible presence or absence of amorphous silica. According to previous studies [21, 59, 63, 64], if quartz luminesces red, it has been heated or melted and then recrystallized but does not contain amorphous silica. In addition, tectonic deformation lamellae may appear red but not black [21, 59, 63, 64]. Non-shocked quartz lattice often luminesces blue under CL [21, 59, 63, 64].
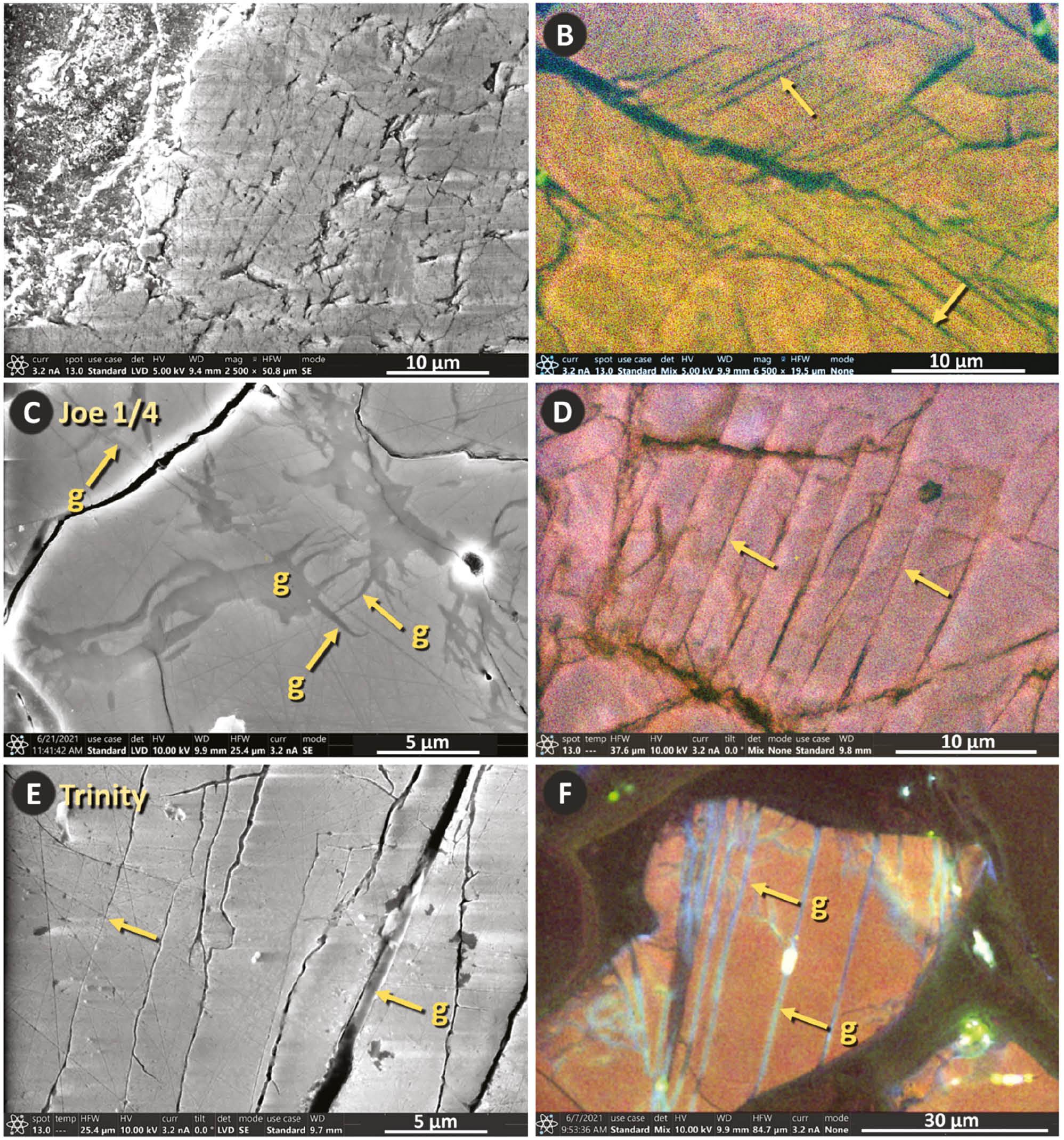
SEM (A-C) images and cathodoluminescence (CL) images (D-F) of shock fractures in quartz grains. (A) SEM-BSE image of quartz from Meteor Crater, grain 11x08. Shock fractures at arrows. (B) CL image of a different Meteor Crater grain 13x11 showing small, feather-like fractures angling away from the large irregular shock fracture. (C) SEM-BSE image from the Joe-1/4 site, grain 03x16. Most shock fractures contain darker-contrast glass (g) along the shock fractures. The web-like structure is consistent with the high-pressure injection of molten silica or in situ melting. (D) CL image of a different grain from the Joe-1/4 site, grain 14x-04b. (E) SEM-BSE image of quartz from the Trinity site, grain 09x11. The arrow at “g” marks non-luminescent glass. (F) CL image of a different grain, 06x14, from Trinity meltglass. Note that shock fractures are filled with bluish-gray-to-black, non-luminescent glass.
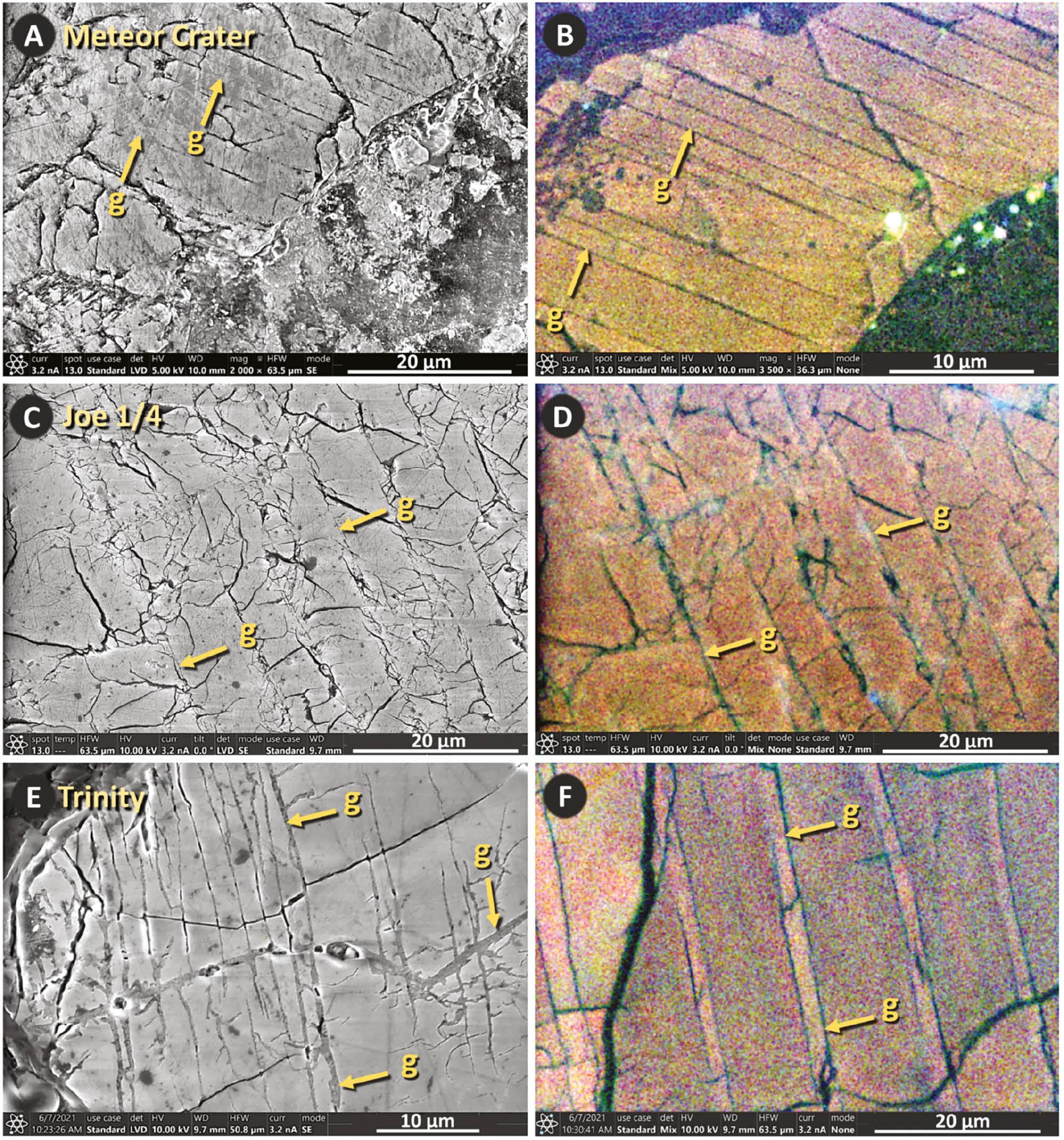
SEM and cathodoluminescence (CL) images of shock fractures in quartz. (A, B) Grain 14x-04a from Meteor Crater, Arizona. (C, D) Grain 09x14 from the Russian Joe-1/4 nuclear test. (E, F) Grain 32x08 from Trinity meltglass. The red arrows point to sub-parallel pairs of shock fractures in the SEM-BSE images (left-hand column) and CL images (right-hand column). In SEM-BSE images (left-hand column), yellow arrows point to thin, dark-gray bands of amorphous silica labeled “g.” In the CL images (right-hand column), the bluish-gray-to-black bands at arrows labeled “g” indicate non-luminescent, glass-filled shock fractures. As confirmed by EDS, the material is amorphous silica (glass).
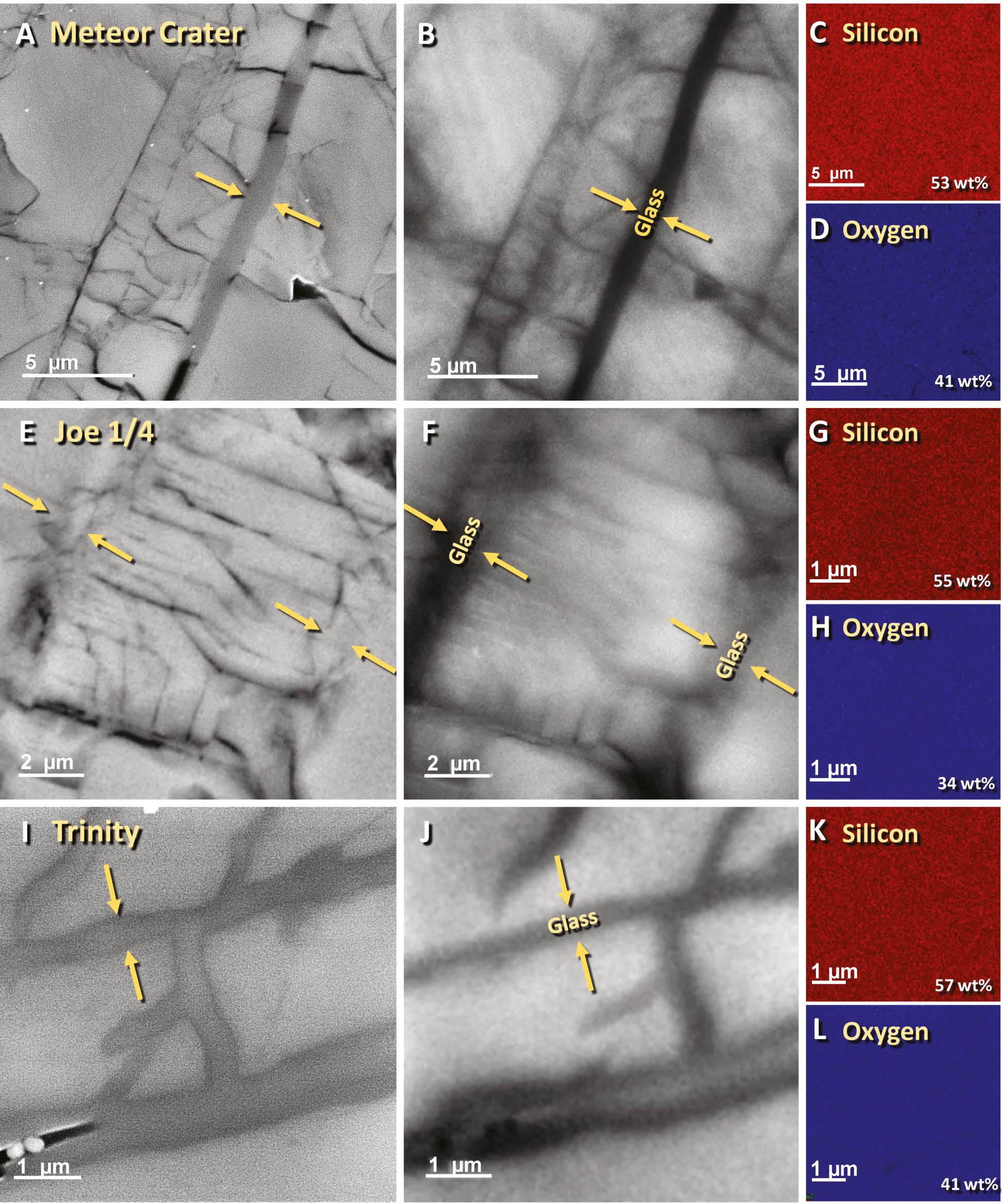
Images acquired using SEM, grayscale panchromatic cathodoluminescence (CL), and energy dispersive spectroscopy (EDS). (A-D) Grain #10x-12 from Meteor Crater, Arizona. (E-H) Grain #14x-04B from the Russian Joe-1/4 nuclear test. (I-L) Grain #32x08 from Trinity meltglass. In the SEM-BSE images (left-hand column), the yellow arrows point to shock fractures filled with gray material. In the grayscale panchromatic CL images (spectrum: 185–850 nm; middle column), the yellow arrows point to the corresponding region, marked as glass. The gray-to-black color indicates that the filling material is non-luminescent, consistent with amorphous silica [21, 59, 63, 64]. The SEM-EDS panels (right-hand column) are of approximately the same field of view as in the left-hand column and confirm that the material is predominantly composed of silicon and oxygen (see EDS spectra for panels in Appendix, Figures S8–S12). Thus, the evidence indicates that the filling in the fractures is amorphous silica.
SEM energy dispersive spectroscopy (SEM-EDS)
For these analyses, we selected multiple areas that displayed fractures filled with material (Figures 7 and 8). In most cases, EDS analyses indicated the quartz matrix and filling material were predominantly silica and oxygen (range: 89–98 wt%). The balance was made up of carbon, presumably from the mounting epoxy or the carbon coating. For EDS spectra and other details, see Appendix, Figures S8–S12.
Electron backscatter diffraction (EBSD)
Analyses performed using EBSD rely on varying comparisons of the Kikuchi patterns in a given grain, as shown in Appendix, Figures S13–S16. Multiple EBSD routines reveal an extensive network of oriented shock fractures for all three sites (Figure 12). Optical microscopy revealed that most of the hundreds of quartz grains in each sample from the three sites display these fractures. These images closely match those from shock experiments at ≥5.5 GPa by Kowitz et al. [11] (Figure 1).
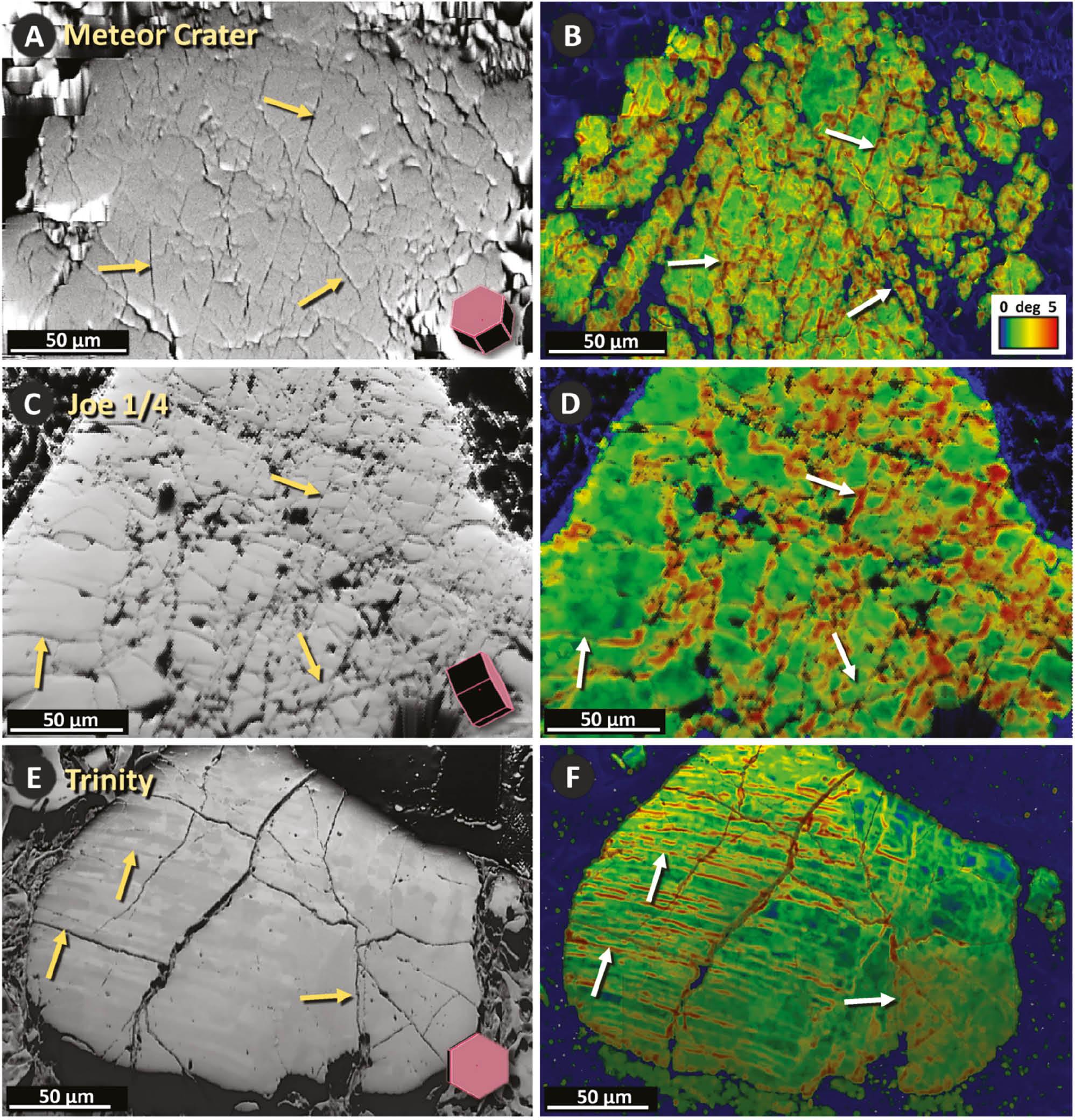
Images of fractures using EBSD. (A, B) Grain #10x-12 from Meteor Crater, Arizona. (C, D) Grain #14x-04B from the Russian Joe-1/4 nuclear test. (E, F) Grain #09x11 from Trinity meltglass. Images in the left-hand column show numerous oriented shock fractures, with arrows marking a few representative examples among the many fractures present. For a close-up view of the smaller fractures, see SEM-BSE image Figure 9E. For reference, the crystal representation at the lower right-hand of each image (left-hand column) represents the crystallographic orientation of that grain in which the c-axis is perpendicular to the red basal plane. A multi-colored misorientation scale is inset into the lower right-hand of panel B and applies to all images in the right-hand column. The colors represent the degrees of misorientation of the crystalline structure, ranging from 0 degrees (blue) to ~5 degrees (red). Note that the largest misorientation (i.e., damage) is concentrated along shock fractures. Some apparent disorientation might be an artifact of weaker quality diffraction patterns in the amorphous material or is due to surface irregularities near fractures, causing locally noisier orientation data.
Each grain’s crystallographic orientation is indicated for each image in the left-hand column by the crystal representation in the lower right-hand corner (Figures 12A, 12C, and 12E). The red-colored plane represents (0001), the basal plane, with the c-axis perpendicular. Although the shock fractures are non-planar, their general orientations correspond well with the crystallographic planes depicted on the crystal representation in the lower right-hand corner. This correspondence suggests that the shock fractures form similarly to high-shock planar deformation features (PDFs) and planar fractures (PFs) but are unlike tectonically-deformed lamellae [8, 66].
EBSD “local orientation spread” (LOS)
The high pressures during shock metamorphism damage and distort the crystalline lattice of quartz grains. To identify and quantify any potential grain damage, we used an EBSD routine called “local orientation spread” that generates Kikuchi patterns of the quartz lattice. The EDAX EBSD software compares these short-range patterns to reveal possible rotations or misorientations of the crystalline lattice, after which the average misorientation of any given point is calculated relative to neighboring points. For the three sites, we observed values ranging from 0° to ~5° of misorientation, and this misoriented lattice tends to be concentrated along the shock fractures (Figure 12). We found that such misorientations are common in quartz with shock fractures, but are atypical in unshocked quartz grains (e.g., Figure 16).
Trinity grain 32x08 was scanned using SEM (Figure 13A) that recorded EBSD data with a beam width of ~20 nm and indexed the crystallographic patterns automatically (Figure 13B). This provides information about the orientation of the crystal at that spot relative to sample coordinates, generally defined by three Euler angles that relate sample and crystal coordinate systems. Figure 13C is a map over the same Trinity quartz grain with colors indicative of Euler angle f2; the Kikuchi pattern is shown in Figure 13D. The pole figure in Figure 13B shows that two main orientations are present across the selected area. The quartz grain has a c-axis roughly perpendicular to the sample surface (001 pole figure) and two orientations of rhombohedral planes (101 and 011) related by a 60° (180°) rotation around the c-axis. This orientation relationship is known as Dauphiné twinning, which can form in multiple ways: during growth, during the phase transition from hexagonal high quartz to trigonal low quartz, during mechanical deformation, or during recrystallization after thermal shock. Several studies have observed Dauphiné twins in quartz subjected to stress (e.g., Schubnikow and Zinserling [67]; Tullis [68]; and Wenk et al. [41]). From the Euler angle relationships, twin boundaries can be defined, and the Dauphiné twin boundaries are plotted with black outlines in Figure 13C.
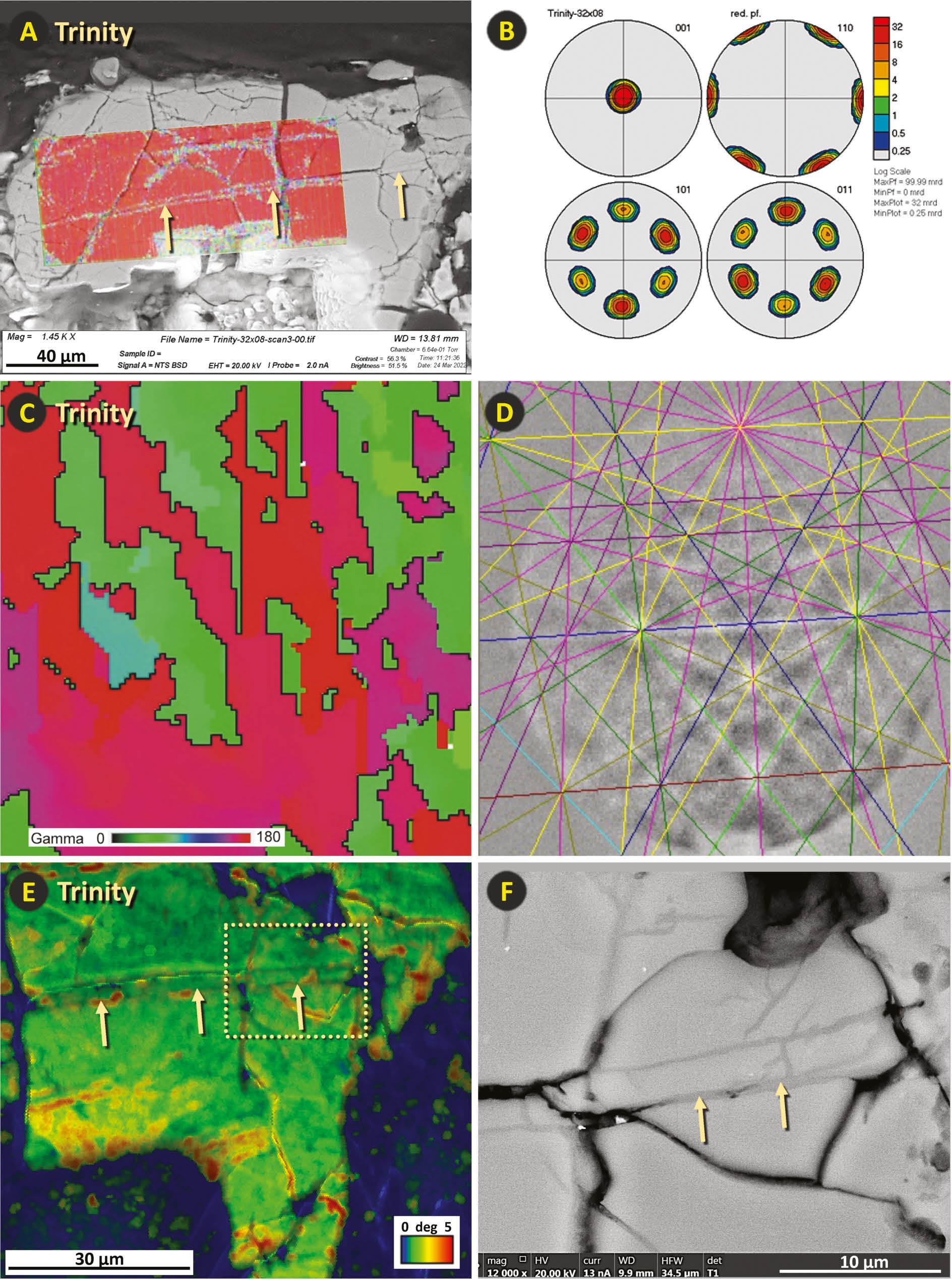
Images of selected portions of shock-fractured quartz grain 32x08 from Trinity meltglass. (A) EBSD “image quality” scan in red is superimposed on an SEM-BSE image; arrows mark a pair of oriented, sub-parallel shock fractures with damaged lattice, as indicated by the lack of the red EBSD signal. (B) Pole figures across the grain with the c-axis (0001) nearly perpendicular to the surface but with Dauphiné twins that share two orientations rotated 60 degrees (101 and 011). (C) EBSD map of Euler angle gamma displays mainly two orientations (green and red). They are related by Dauphiné twinning (180 – 30 deg rotation around the c-axis, black outlines). Equal area projection. (D) Kikuchi patterns corresponding to EBSD scan in panel C. (E) Image quality and local orientation spread (LOS) image of lattice misorientations (yellow to red) that correspond to the sub-parallel shock fractures at arrows. (F) Close-up SEM-BSE image of oriented shock fractures, marked by gold-dotted box in panel E. Medium gray areas represent amorphous silica, as separately confirmed by SAD, FFT, and TEM-EDS.
EBSD “grain reference orientation deviation” values superimposed on EBSD “image quality” values
Orientation deviation maps (Figure 14) assist with visualizing the distribution of local lattice angular misorientations by color-coding the variations. EDAX’s EBSD software analyzes and colorizes individual points to illustrate any rotation of the crystalline lattice around an arbitrary common point on the grain with a wide range of colors that each represents areas with short-range misorientations relative to the common point.
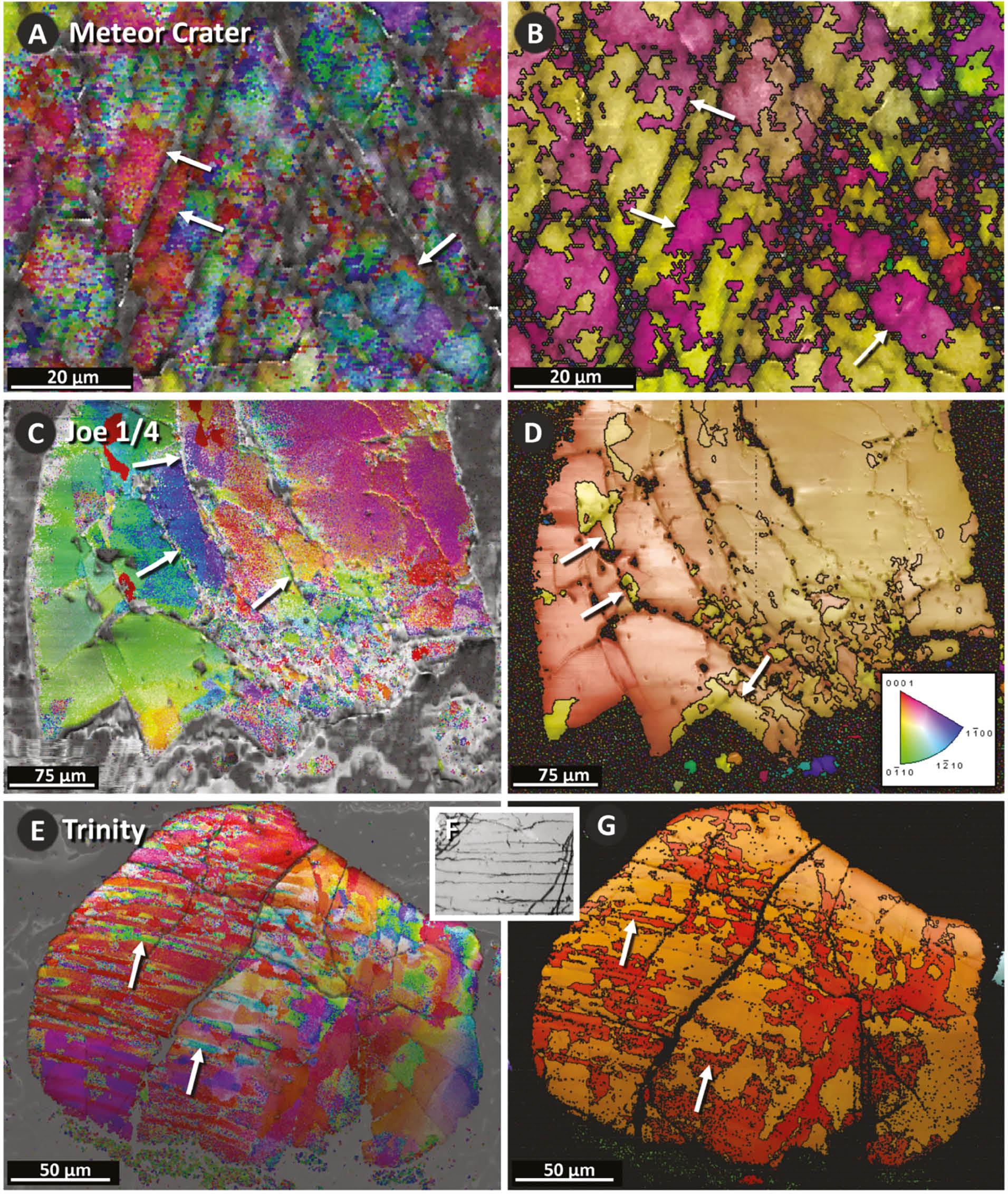
EBSD images using “orientation deviation” superimposed on “image quality” and EBSD “inverse pole figure” superimposed on “image quality.” (A, B) Grain #10x-12 from Meteor Crater, Arizona. (C, D) Grain #19x-12C from the Russian Joe-1/4 nuclear test. (E-G) Grain #09x11 from Trinity meltglass. (A, C, E in the left-hand column) Orientation deviation analyses show the crystalline misorientation of the grain relative to an average value. Note that the misorientations tend to align with shock fractures (gray-to-black colored) at the white arrows. (F) Epi-illumination image showing open fractures corresponding to arrow in panel C. (B, D, G in right-hand column) Inverse pole figure analyses illustrate the axes of rotation of areas around the c-axis. In each figure, the white arrows mark black-outlined Dauphiné twins that are rotated 60° around the c-axis of these monocrystalline quartz grains. This twinning is represented by the magenta color in panel B, yellow color in D, and red in G. Note that most Dauphiné twins are oriented along shock fractures (gray-to-black colored), suggesting that the twinning formed synchronously with the shock fractures and is common in all quartz grains from the three sites investigated here. The inset legend in panel D shows the color-coded Miller-Bravais crystalline axes for all six panels.
Several of the grains in Figure 14 exhibit shock fractures that are curved. As the shock fractures formed, the lattice may have become distorted at high ambient temperatures or by shock melting, as suggested by Buchanan and Reimold [16] and Reimold and Koeberl [13].
EBSD “inverse pole figure” values superimposed on EBSD “image quality” values
The inverse pole figures (right-hand column of Figure 14) reveal variations in the lattice axes of quartz relative to a frame of reference, which, in these examples, is the (0001) basal plane. The EBSD results indicate that these are monocrystalline grains. In each case, measurements show that areas of quartz grains known as Dauphiné twins are rotated 60° relative to the c-axis. Dauphiné twinning is undetectable by standard optical microscopy and SEM but can easily be seen using EBSD.
For the shocked quartz analyzed in our study, Dauphiné twins typically align with the trend of the shock fractures, suggesting that they crystallized as the fractures formed under high stress or formed after the grain fractured as it cooled from the high shock temperatures. It has long been recognized that Dauphiné twins form when quartz is subjected to mechanical stress [67]. Later, Wenk et al. [41] further concluded that Dauphiné twinning occurs under high thermal and mechanical stress. Subsequently, Wenk et al. [42] reported that Dauphiné twinning provides evidence for an impact-related origin of shocked quartzite collected from the Vredefort crater in South Africa.
Natural and tectonically deformed quartz grains
This study used optical microscopy and SEM-EDS to investigate hundreds of HF-etched natural, unshocked quartz grains and tectonically deformed grains. These grains commonly displayed fractures, but SEM-EDS observed none to contain silica. In addition, although Dauphiné twins are nearly ubiquitous in all quartz grains, including unshocked or tectonically deformed grains, they are typically distributed randomly (Figures 15 and 16). This random distribution is unlike shock-fractured quartz grains in which Dauphiné twins are nearly always oriented with the fractures.
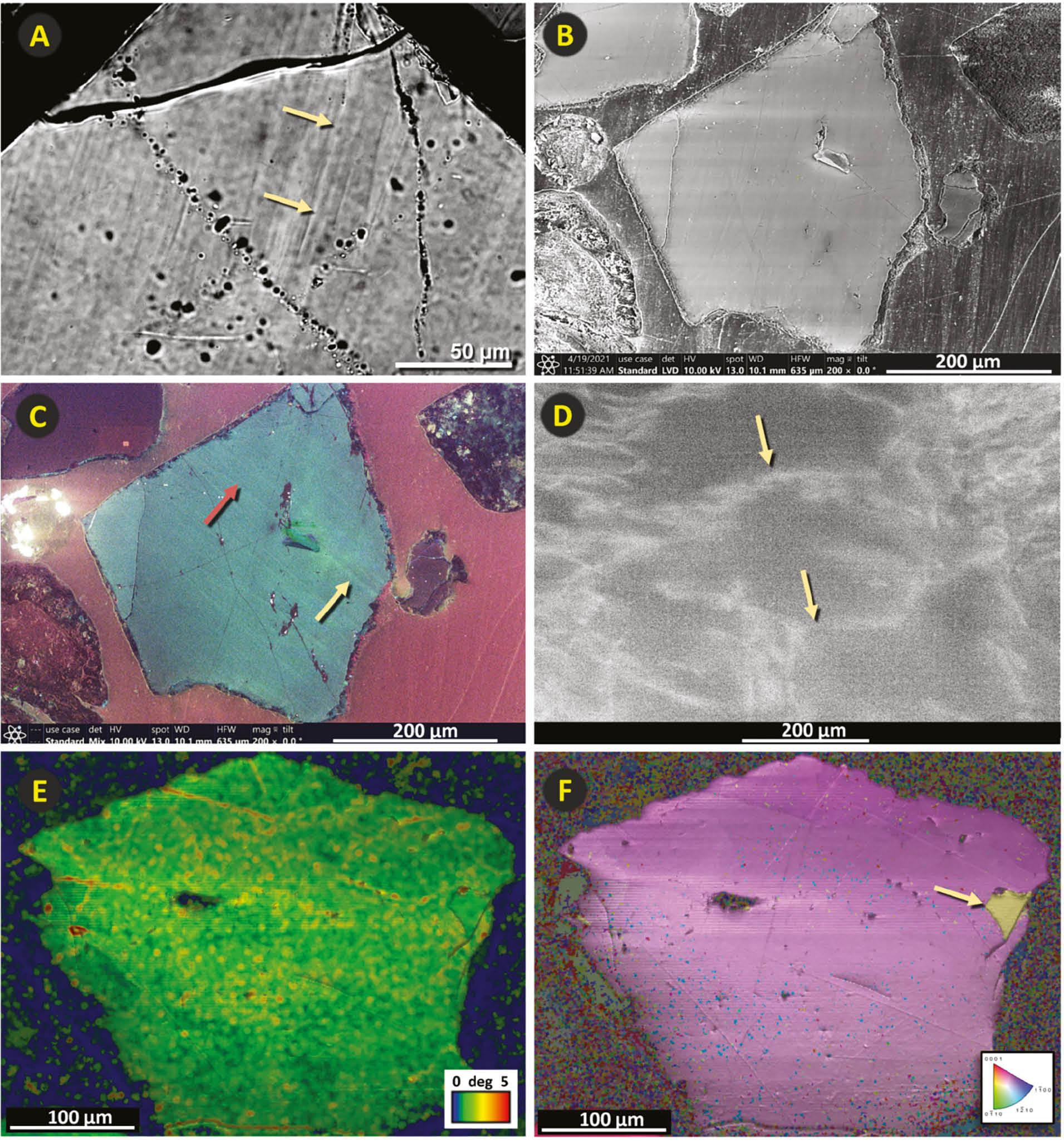
Tectonically-deformed quartz from a non-impact site in Syria. (A) Optical microscopy image shows tectonically-deformed lamellae, marked by yellow arrows. (B) SEM-BSE image: tectonic lamellae are not visible on the surface. (C) Cathodoluminescence (CL). The tectonic lamellae are faintly visible as blue streaks in the grain. Blue luminescence indicates that the quartz is natural and unshocked [21, 59, 63, 64]. The red arrow marks the extraction location of the ion beam (FIB) foil for use with TEM. (D) Bright-field TEM image with no parallel lamellae; yellow arrows mark irregular areas characteristic of dislocations in the quartz. (E) EBSD image quality (IQ) and local orientation spread (LOS) image shows no significantly aligned misorientations. (F) EBSD IQ superimposed on inverse pole figure (IPF); the single Dauphiné twin (yellow arrow) is not oriented with any features in the grain, except the single fracture to the right. In this grain, the tectonic lamellae are only visible in the optical and CL images and not in other analyses, as they are in shock fractures. These multiple techniques enable differentiation between non-shock tectonic lamellae and impact-related shock fractures.
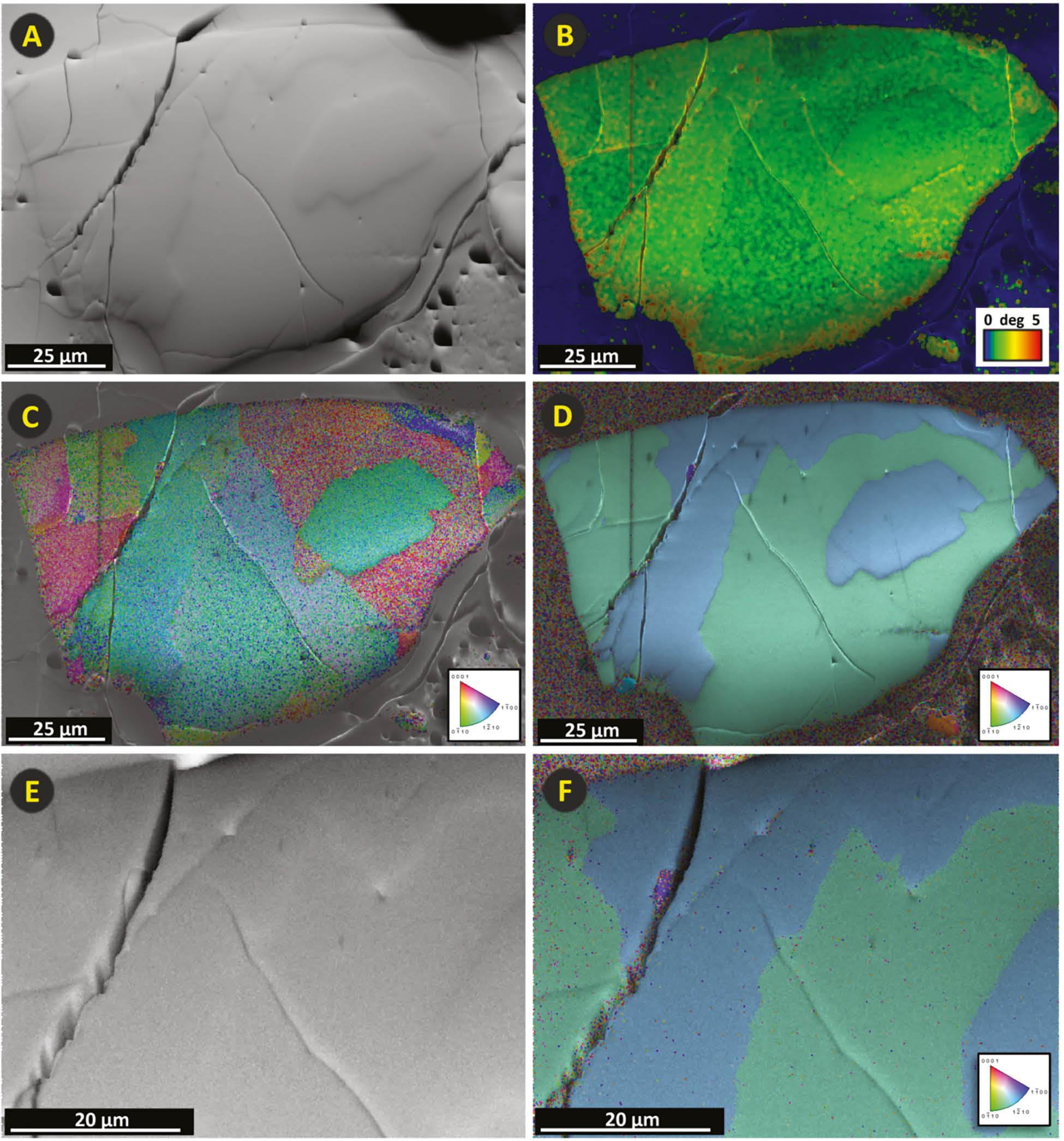
Natural, fractured, and unshocked quartz from the Russian Joe-1/4 site. (A) The EBSD image shows a few fractures, but they are not glass-filled. (B) EBSD image quality superimposed on local orientation spread shows no shock fractures aligned with locally misoriented lattice. (C) EBSD image quality (IQ) and grain reference orientation deviation (GROD) show no pattern of misoriented lattice compared to the grain’s average orientation. (D) EBSD image quality (IQ) and inverse pole figures (IPF) illustrate variations in the lattice axes of quartz relative to a chosen crystal reference frame, which for these grains is the (0001) basal plane. These color variations represent Dauphiné twinning (blue and green) but are not oriented along the fractures. (E) Close-up SEM-BSE image of quartz grain. (F) SEM-BSE and EBSD inverse pole figures. This grain is fractured, but the fractures are not oriented as in shock fractures. In addition, no amorphous silica was found associated with the lamellae. No well-oriented lamellae are visible in any of these images.
Additional imagery showing variations in shock-fractured quartz
Given the importance of imagery for this investigation, we provide additional examples from Trinity, Joe, and Meteor Crater (Figures 17–19). These illustrate the wide variation in shock fracture characteristics that we documented. These images were acquired using the same analytical techniques presented above.
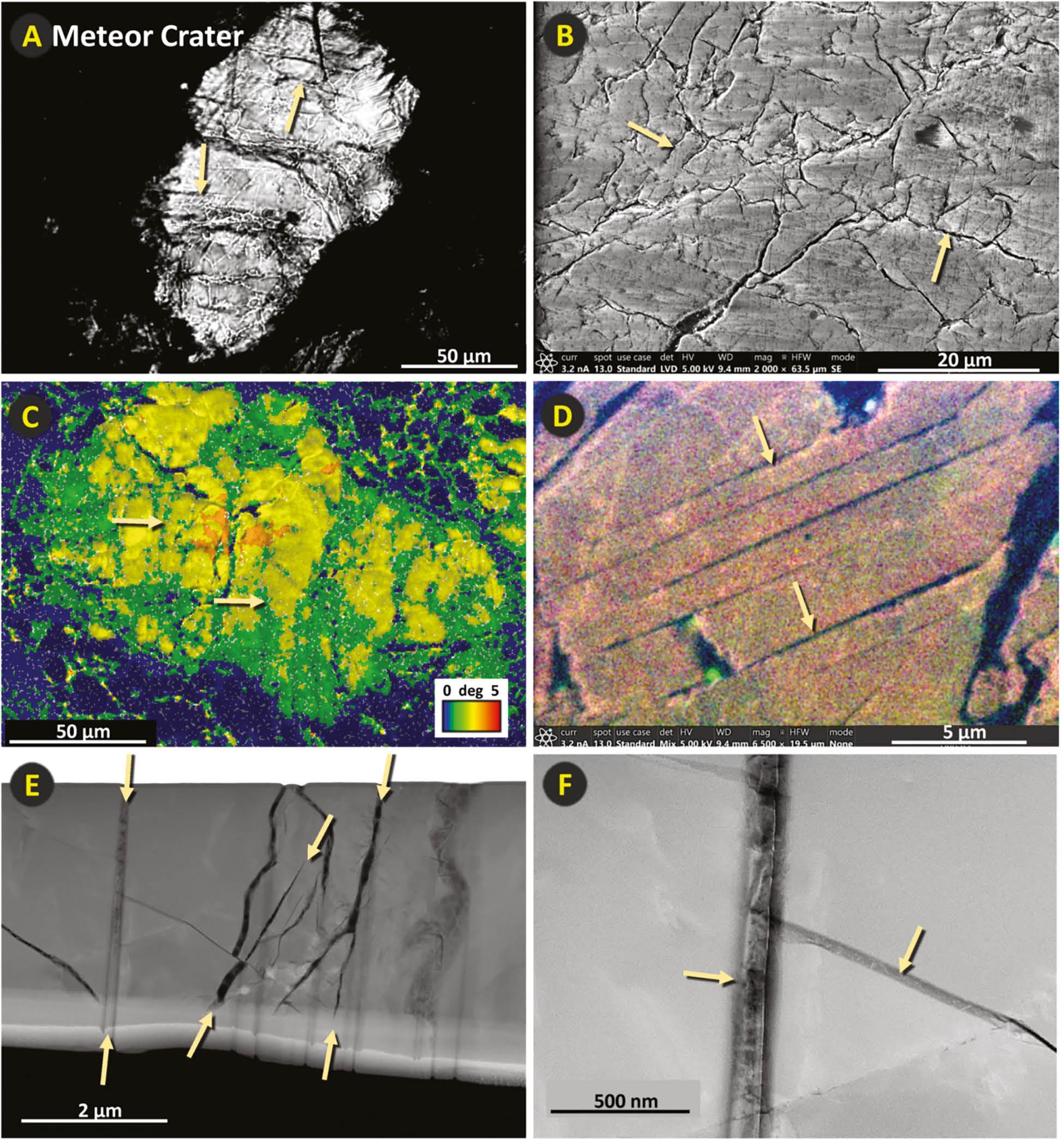
Images of selected portions of shock-fractured quartz grain 09x-11 from Meteor Crater. (A) Optical photomicrograph. Arrows mark selected shock fractures. (B) SEM-BSE image. (C) EBSD image quality (IQ) and grain average image quality (GAIQ). Green areas at the arrows represent areas that correspond with shock fractures. (D) Cathodoluminescence (CL) image of non-luminescent gray-to-black areas at arrows indicating amorphous silica in areas corresponding to oriented shock fractures. (E) Bright-field TEM image of open, glass-filled shock fractures. (F) Close-up TEM image of glass-filled shock fractures.
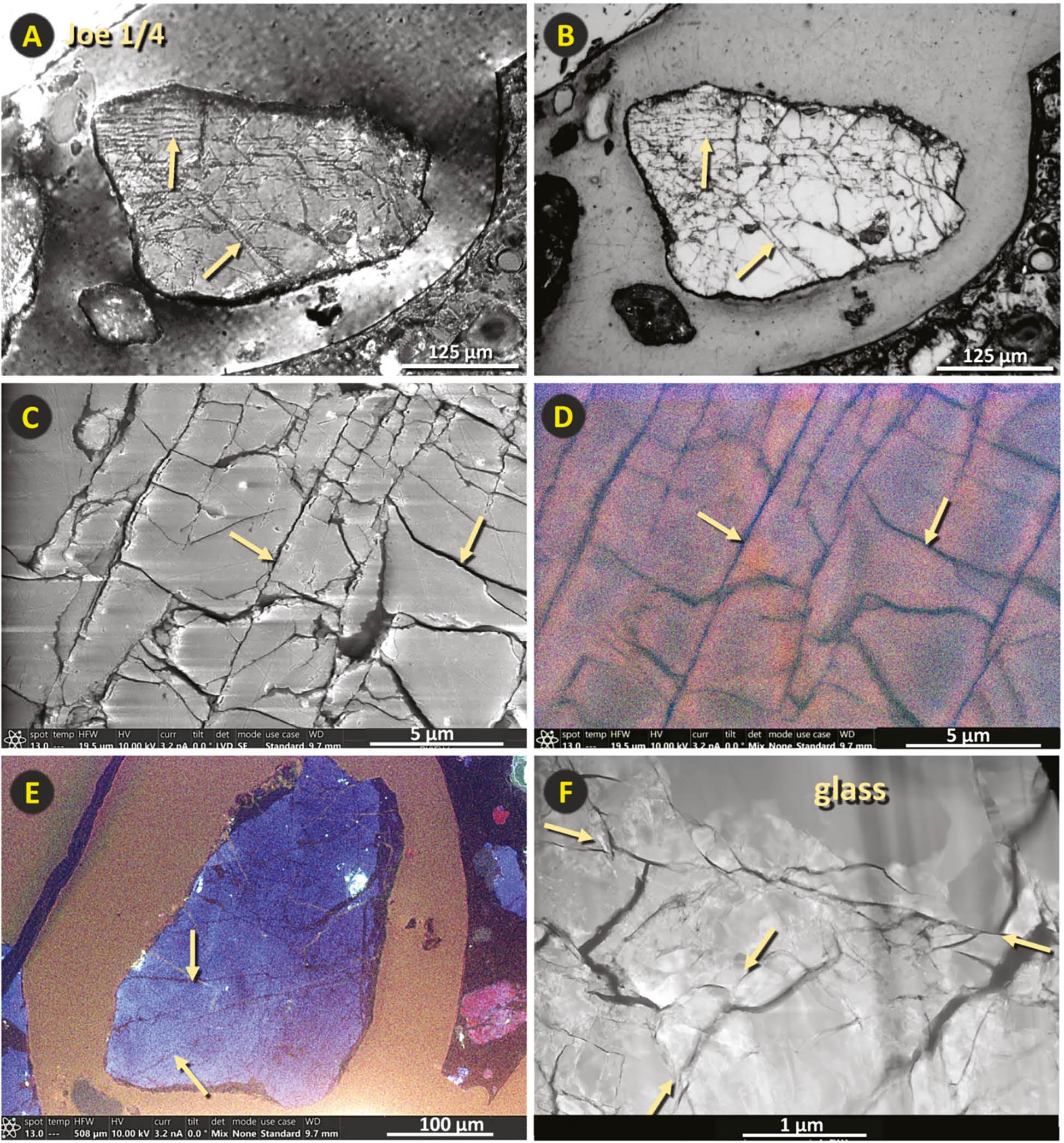
Images of selected portions of shock-fractured quartz grain 12x12 from the Joe-1/4 atomic test site. (A) Optical photomicrograph with arrows pointing to selected shock fractures. Yellow arrows mark fractures. (B) EPI photomicrograph of the same view as panel A. (C) SEM-BSE image. (D) Cathodoluminescence (CL) image shows non-luminescent black lines at arrows indicative of amorphous silica, as confirmed by TEM in panel F. Approximately the same view as in panel C. (E) Cathodoluminescence (CL) image shows blue-colored, unshocked quartz matrix containing non-luminescent black lines at arrows indicative of amorphous silica along shock fractures. (F) TEM image of oriented and unoriented shock fractures. The notation “glass” marks a darker gray subrounded area composed of amorphous silica.
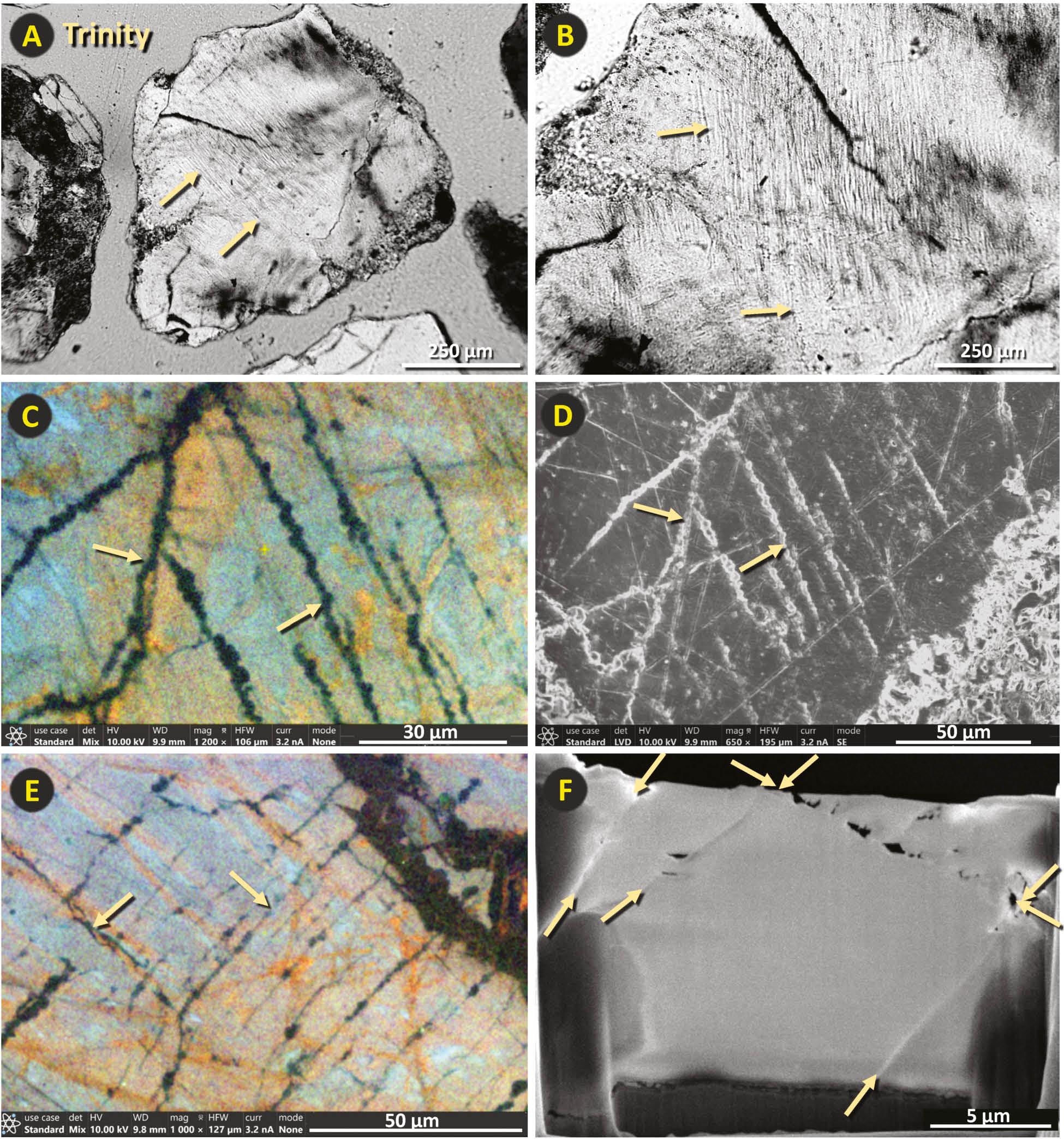
Images of selected portions of shock-fractured quartz grain 30x08 from Trinity JIE grains sample. (A) Optical photomicrograph of selected shock fractures at arrows. (B) Close-up optical photomicrograph. (C) Cathodoluminescence (CL) image of non-luminescent, black lines at arrows indicative of amorphous silica associated with shock fractures, as confirmed by TEM. (D) SEM-BSE image of approximately the same grain region as shown in panel C. (E) Another CL image of non-luminescent, black lines indicates the presence of amorphous silica. (F) TEM image with arrows marking three directions of shock fractures.
Potential formation mechanisms of shock fractures
This investigation supports the hypothesis that glass-filled shock fracturing can occur in nuclear detonations and crater-forming impact events. Although the characteristics of these two events are mostly dissimilar, there are essential similarities in the shock effects. Both events produce enormous temperatures and pressures capable of melting quartz and producing shock metamorphism. The most important similarity is that, in both events, the fireball’s shockwave is coupled with Earth’s surface. This situation is unlike high-altitude nuclear detonations in which the fireball does not intersect the Earth’s surface. This coupling appears essential for providing the following mechanisms to produce amorphous silica in shocked fractures.
Shock fracturing by compression
Evidence indicates that shock fractures, as well as shock PDFs and PFs, form when quartz grains are subjected to shock pressures above their Hugoniot elastic limit (HEL), which, for quartz, ranges from ~3–15 GPa [27]. This pressure range corresponds with that estimated for the nuclear tests of Trinity and Joe-1/4. A pressure database [69] reveals that in quartz, velocities of the pressure wave range from 6.3 to 6.9 km/sec, depending on a quartz grain’s orientation.
High shock pressures commonly produce quartz phases called coesite or stishovite. However, we found no evidence of these phases previously observed at Meteor Crater [2, 3, 32, 48, 49, 70–73]. The absence of these phases supports the hypothesis that the shocked grains investigated in this study from Trinity, Joe, and Meteor Crater formed at the lower range of shock pressures, estimated to be ≤8 GPa.
Shock fracturing by tension
In both airbursts and crater-forming events, the fracturing of quartz grains may also occur from tensile forces and spallation [26, 35, 37, 74–76]. This shock occurs when a compressive shockwave enters a material, such as a quartz grain, and then reflects off the opposite grain boundary, producing a rarefaction wave that fractures the grain in the opposite direction. The shockwave may occur at < 1 GPa and does not need to exceed quartz’s Hugoniot elastic limit (HEL) to cause tensile damage. This process frequently produces the most mechanical damage because the tensile strength of quartz is typically lower than its compressive strength. In this study, tensile fracturing is considered to be the most common formation process.
Thermal shock-metamorphism
For shock fractures to form in quartz, the crystalline lattice must experience high stress and strain, not just from high pressures but also typically from high-temperature gradients. Nuclear tests like Trinity generate fireballs with extreme temperatures that may rise to ~200,000 °C within 10−4 sec but then, after 3 sec, drop to below the melting point of quartz [77]. Such extreme, short-lived temperatures followed by rapid quenching can fracture quartz grains due to sudden thermal expansion followed by rapid cooling. In addition, the intense thermal and gamma radiation may heat the quartz grains to near-melting and, thus, reduce the pressures needed to form shock fractures. These thermal processes appear responsible for forming Dauphiné twinning in alignment with the shock fractures. To our knowledge, this is the first report of such a connection.
Most importantly, we concur with the jetting hypothesis by Kieffer [32] that high temperatures appear to vaporize quartz grains and sediment, after which high pressures inject molten silica or vapor into the fractures and any other zones of weakness in exposed quartz grains [33, 37]. We infer that molten silica might enter quartz grains along multiple possible zones of weakness: (i) fractures produced by the shockwave; (ii) fractures produced by high temperatures; (iii) pre-existing quartz fractures; (iv) new fractures that form along pre-existing PDFs and PFs; (v) new fractures along pre-existing tectonic lamellae; and (vi) new fractures along pre-existing subgrain boundaries. In the cases of the pre-existing features, the shock fracturing process overprints and modifies the existing features. Even though these types of fractures may form under substantially different shock and non-shock conditions, all have one common characteristic: they became filled with amorphous silica, as described next.
Previous studies of amorphous silica in quartz grains
There have been many studies that identified amorphous silica in quartz. Kieffer [32] analyzed shocked sandstone from Meteor Crater and concluded that impact-related glass-filled fractures began to form at 5.5 GPa but not at lower pressures (Table 2). Christie and Ardell [18] performed shock compression experiments on large quartz cylindrical crystals and noted amorphous silica that filled the fractures at a confining pressure of 1.5 GPa. Kenkmann et al. [78] performed shock experiments on 1.5-mm-wide cylindrical samples of quartz, and using moderate shock pressures of 6–34 GPa, they could generate veins of amorphous silica that were 1–6 μm wide. Kowitz et al. [11, 15, 46] conducted detailed laboratory experiments to determine the lower pressure limit for forming shock features called “sub-planar, intra-granular fractures.” [11] In their experiments, a steel plate was explosively driven into cylinders of quartz-rich sandstone at pressures of 5, 7.5, 10, and 12.5 GPa. Visible shock fractures and amorphous silica (~1.6 wt%) first appeared at 5 GPa [11], similar to the results of Kieffer [32]. Carl et al. [7] conducted experiments demonstrating that extensive amorphization of quartz begins at ~10 GPa. In quartz grains experimentally shocked at 5 to 17.5 GPa, Fazio et al. [5] observed glass veins composed of amorphous silica generally thicker than 50 nm, extending several microns in length. Wilk et al. [6] found amorphous silica in experimentally shocked rocks called shatter cones that formed at low shock pressures of 0.5–5 GPa. Laboratory shock experiments by Martinelli et al. [79] used quartz crystals with a minimum diameter of 3400 μm, larger than we tested. The reported compression applied was as low as 0.2 GPa; the maximum compression applied is unclear but appears to have been <1 GPa.
In summary, these studies report the formation of amorphous silica in fractures produced by minimum shock pressures averaging 4.2 GPa (range 0.2 to 10 GPa), with 5 of the 8 studies reporting ~5 GPa as the minimum observed pressure. No experimental study has ever reported glass-filled fractures in natural quartz grains, nor have they been reported in natural quartz grains exposed to non-impact processes, such as volcanism and tectonism [19, 80]. The existing evidence supports their formation during nuclear detonations and hypervelocity impact events. In addition, Ernstson et al. [26, 34, 36, 37, 76, 81, 82], Moore et al. [83], Demitroff et al. [84], and Mahaney et al. [85] have reported shock-metamorphosed quartz in multiple proposed airbursts during the Cenozoic.
Proposed model for producing shock fractures
To summarize, we propose that shock fractures form in the following sequence. (i) Fractures in quartz grains either pre-exist or are produced by the high-pressure shockwave and thermal pulse both by compression and tensioning; (ii) the blast vaporizes some quartz grains, and this vapor is transported away from ground zero in the expanding fireball; (iii) the outer surfaces of some quartz grains melt at >1720 °C, the melting point of quartz; (iv) the extreme pressures inject molten silica or silica vapor into the fractures; and (v) both thermal and pressure shock may cause further random melting on the exteriors and in the interiors of some grains.
Future studies
Several studies [34, 36, 37, 75, 86] have reported evidence that shock fractures are produced in cosmic airbursts when a high-pressure, high-temperature fireball intersects the surface, similar to the nuclear airbursts described here. These cosmic airbursts may produce shallow craters rather than classic hard-impact craters. We suggest that future studies investigate the hypothesis that low-shock, glass-filled shock fractures are produced in quartz grains during near-surface cosmic airbursts. Similarly, we suggest further research to improve our understanding of glass-filled fractures in hard-impact craters of all sizes.
Conclusions
Glass-filled shock lamellae and fractures are considered to be definitive indicators of a crater-forming impact event and are widely accepted to form at extreme pressures of ~5 to >30 GPa. However, most previous studies of shocked quartz were conducted on large craters and on easily recognizable quartz grains that had been shocked at the higher end of that pressure range. Consequently, there is limited knowledge about the characteristics of quartz grains minimally shocked at lower shock pressures.
This study confirmed previously reported low-shock fractures in quartz at Meteor Crater, a relatively small 1.2-km-wide impact event. Most importantly, we confirmed that similar low-shock fractures also form in near-surface nuclear airbursts, where the fireball and the blast wave reach the surface and no hard-impact crater forms. Despite being static instead of moving at high velocity, these nuclear airbursts create ambient conditions of high pressures and temperatures that are proposed to be similar to those near-surface cosmic airbursts in which the shockwave couples to Earth’s surface.
We observed that these low-grade shock fractures: (i) are either void or filled with glass; (ii) range from near-planar to curvilinear; (iii) are commonly sub-parallel in orientation; (iv) are commonly spaced microns apart; (v) are typically less than one micron thick; (vii) are typically closely aligned with Dauphiné twins; and (viii) appear to form at <5 GPa. Notably, Dauphiné twinning occurs during exposure to high pressures or high temperatures, after which portions of the grains recrystallize in alignment with the fracture patterns. Multiple studies have concluded that when amorphous silica is present within fractures, it allows for the unequivocal differentiation between impact-related shock fractures and the glass-free lamellae that mark slow-strain tectonic deformation. The same principle applies to shock fractures formed in nuclear detonations. Thus, we conclude that these shock fractures cannot be of tectonic origin.
The discovery of shock fractures in quartz exposed to nuclear airbursts has important implications. It suggests that shock metamorphism may also occur during a near-surface airburst of an asteroid or comet if the bolide disintegrates close enough to the Earth’s surface to generate large shock pressures. The protocol reported here may help identify low-shock fractures in quartz from previously unknown, near-surface cosmic airbursts and small crater-forming impact events in the past.